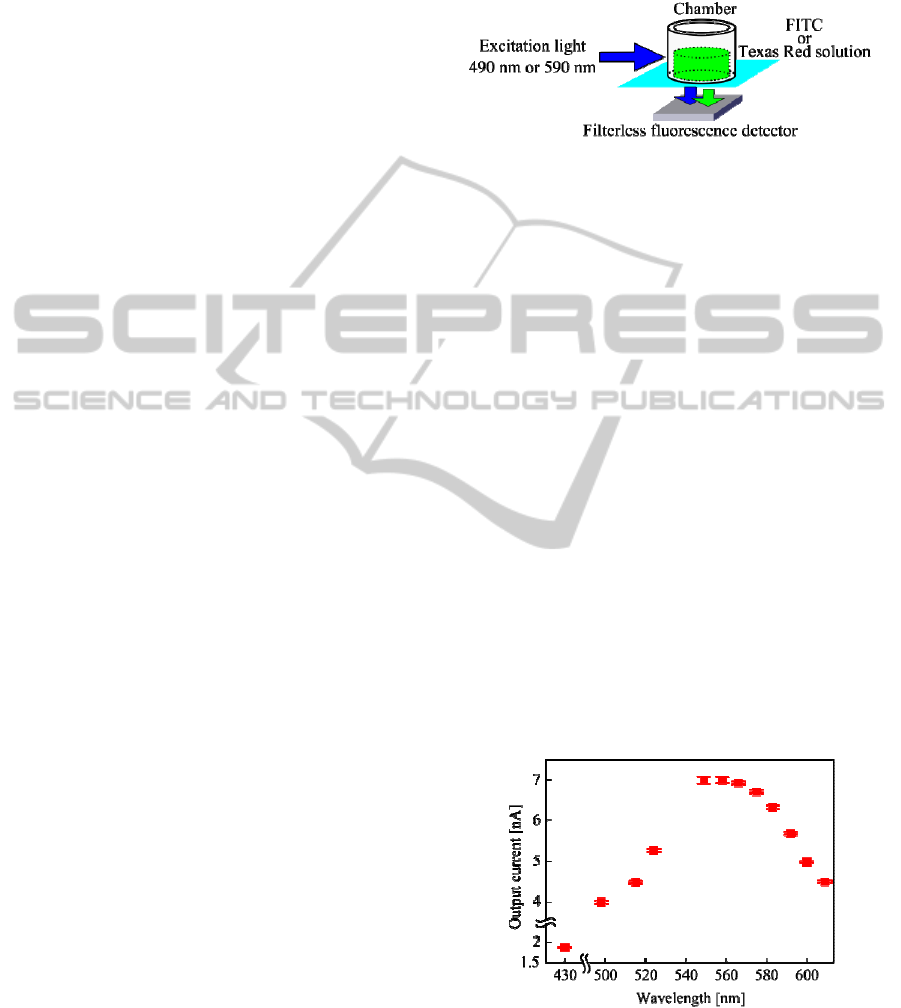
(8230E, ADC Corporation) to keep fixed light pow-
er of 100 μW/cm
2
for the reference.
3.1.2 Detection Limit
On the assumption of FITC use, the sensor was
irradiated by commercial light emitting diode (LED)
(L-7113VGC-H, Kingbright Elec. Co., Ltd.) that had
the dominate wavelength of 525 nm and the output
current was measured at 27 °C. Incident light power
was changed from 0.1 to 100 µW/cm
2
confirmed by
an external power meter (8230E, ADC Corporation).
3.1.3 Temperature Dependency
We investigated the influence of dark current which
comes from thermal excitation. The surrounding
temperature of the sensor was set 21, 27, and 35 °C
independently. Then, output currents were measured.
3.2 Fluorescence Detection
3.2.1 Measurements of FITC and Texas Red
To confirm the sensor’s performance of the quantita-
tive measurements for fluorescent dyes, fluorescein
isothiocyanate (FITC) and sulforhodamine 101 acid
chloride (Texas Red) which are typically used bio-
analysis including SNP genotyping, we measured
the excited fluorescence intensity 525 nm of FITC
(Wako Pure Chemical Industries, Ltd.) or 615 nm of
Texas Red (Life Technologies Corporation) 200 μl
solution of 0, 1, 10 μM individually. Each fluores-
cent solution was prepared using diluted ethanol
(50v/v%). And the each sample solution was set in a
cylindrical plastic chamber of a 7 mm inner diameter
and a 10 mm height as shown in Fig. 5. The excita-
tion lights, 490 nm and 590 nm, for FITC and Texas
Red were independently applied by light source
equipment (LAX-C100, Asahi Spectra Co., Ltd.).
The absorption depth of the sensor was varied by
changing of photo-gate voltage. The intensity of the
fluorescence light and excitation light can be calcu-
lated by using equations (1) where λ
1
= 490 nm, α
1
=
20.0×10
5
m
-1
, w
1
= 0.761 µm, and λ
2
= 525 nm, α
2
=
12.7×10
5
m
-1
, w
2
= 1.135 µm were substituted in the
case of FITC use. In the same way, λ
1
= 590 nm, α
1
=
6.39×10
5
m
-1
, w
1
= 0.803 µm, and λ
2
= 615 nm, α
2
=
4.82×10
5
m
-1
, w
2
= 1.135 µm were substituted in the
case of Texas Red use. The results of detected the
light intensities were normalized assuming the inten-
sity was 1.0 that was a value in the case of measur-
ing 10 μM fluorescent solutions. Furthermore, we
evaluated the possibilities of cross talks of two exci-
tation lights, 590 nm and 490 nm, to FITC and Tex-
as Red. Each fluorescent solution was additionally
exposed by ineffective light for excitation (i.e., 590
nm light for FITC and 490 nm for Texas Red).
Figure 5: Schematic view of the measurement setup.
3.2.2 Detection of FITC and Texas Red Mix
As a model sample of a heterozygote in SNP varia-
tions, we additionally measured the 200 μl mixture
solution of equal parts of FITC and Texas Red (net
0.5 μM for each). The results of detected light inten-
sities were also normalized as same as above section
experiment.
4 RESULTS AND DISCUSSION
Figure 6 shows that the correlation between wave-
length and output current of the sensor. The result
means that the sensor has high sensitivity near the
center of 550 nm at least in this wavelength region.
And also, the sensor has almost the same sensitivity
level for emission wavelengths of FITC (525 nm)
and Texas Red (615 nm).
We think that the similarity of the sensor’s out-
put current level of FITC and Texas Red will enable
us to compare them without any other complicated
calibrations in this study.
Figure 6: Wavelength dependency of the sensor's output.
Figure 7 shows the detection current versus the
incident light intensity. Each data point was obtained
by 10 times experiments and the each error bar was
PROPOSAL FOR A FILTERLESS FLUORESCENCE SENSOR FOR SNP GENOTYPING
187