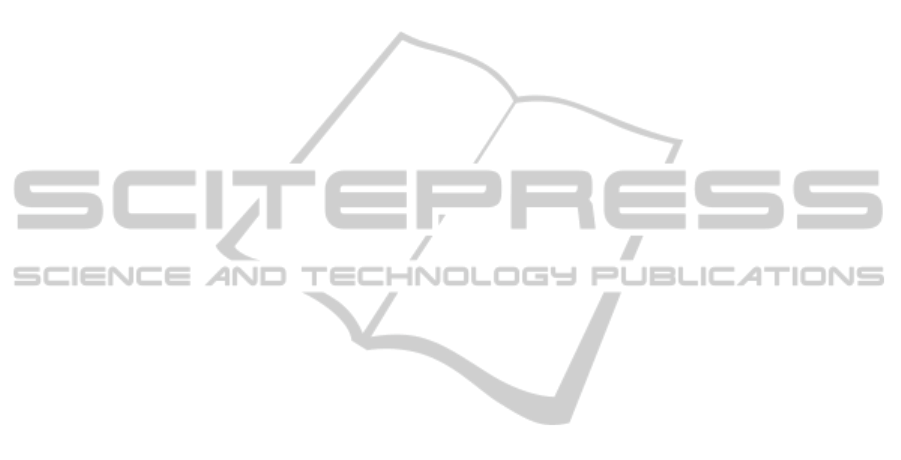
after audio-visual stimulation. Brain, 128 (Pt.12), pp.
2830-2842.
Bolognini, N., Leo, F., Passamonti, C., Stein, B.E., and
Làdavas, E., 2007. Multisensory-mediated auditory
localization. Perception, 36(10), pp.1477–1485.
Bruns, P., Maiworm, M., Röder, B., 2014. Reward
expectation influences audiovisual spatial integration.
Attention, Perception & Psychophysics, [Epub ahead
of print].
Calvert, G., Spence, C., and Stein, B.E., 2004. The
handbook of multisensory processes. MIT Press.
Calvert, G., and Thesen, T., 2004. Multisensory
integration: methodological approaches and emerging
principles in the human brain. Journal of Physiology,
Paris, 98(1-3), pp.191–205.
Cowey, A., 2010. The blindsight saga. Experimental Brain
Research, 200(1), pp.3–24.
Cuppini, C., Magosso, E., Rowland, B., Stein, B., and
Ursino, M., 2012. Hebbian mechanisms help explain
development of multisensory integration in the
superior colliculus: a neural network model.
Biological Cybernetics, 106(11-12), pp.691–713.
Cuppini, C., Magosso, E., Bolognini, N., Vallar, G., and
Ursino, M., 2014. A neurocomputational analysis of
the sound-induced flash illusion. Neuroimage, 92,
pp.248-266.
Driver, J., and Spence, C., 2000. Multisensory perception:
beyond modularity and convergence. Current Biology,
10(20), pp.R731–735.
Foxe, J.J., and Schroeder, C.E., 2005. The case for
feedforward multisensory convergence during early
cortical processing. Neuroreport, 16(5), pp.419–423.
Ghazanfar, A.A., and Schroeder, C.E., 2006. Is neocortex
essentially multisensory? Trends in Cognitive
Sciences, 10(6), pp.278–285.
Isa, T., and Yoshida, M., 2009. Saccade control after V1
lesion revisited. Current Opinion in Neurobiology,
19(6), pp.608–614.
Leo, F., Bolognini, N., Passamonti, C., Stein, B.E., and
Làdavas, E., 2008. Cross-modal localization in
hemianopia: new insights on multisensory integration.
Brain, 131(Pt 3), pp.855–865.
Magosso, E., Cona, F., and Ursino, M., 2013. A neural
network model can explain ventriloquism aftereffect
and its generalization across sound frequencies.
BioMed Research International, 2013, p.475427.
Magosso, E., Cuppini, C., Serino, A., Di Pellegrino, G.,
and Ursino, M., 2008. A theoretical study of
multisensory integration in the superior colliculus by a
neural network model. Neural Networks, 21(6),
pp.817–829.
Magosso, E., Cuppini, C., and Ursino, M., 2012. A neural
network model of ventriloquism effect and aftereffect.
PLoS ONE, 7(8), p.e42503.
Meredith, M.A., and Stein, B.E., 1996. Spatial
determinants of multisensory integration in cat
superior colliculus neurons. Journal of
Neurophysiology
, 75(5), pp.1843–1857.
Passamonti, C., Frissen, I., and Làdavas, E., 2009. Visual
recalibration of auditory spatial perception: two
separate neural circuits for perceptual learning. The
European Journal of Neuroscience, 30(6), pp.1141–
1150.
Sparks, D.L., 1986. Translation of sensory signals into
commands for control of saccadic eye movements:
role of primate superior colliculus. Physiological
Reviews, 66(1), pp.118–171.
Stein, B.E., and Meredith, M.A., 1993. The merging of
senses. Cambridge, MA, The MIT Press.
Tong, F., 2003. Cognitive neuroscience: Primary visual
cortex and visual awareness. Nature Reviews
Neuroscience, 4(3), pp.219–229.
Wallace, M.T., Meredith, M.A., and Stein, B.E., 1993.
Converging influences from visual, auditory, and
somatosensory cortices onto output neurons of the
superior colliculus. Journal of Neurophysiology,
69(6), pp.1797–1809.
ACortico-CollicularModelforMultisensoryIntegration
23