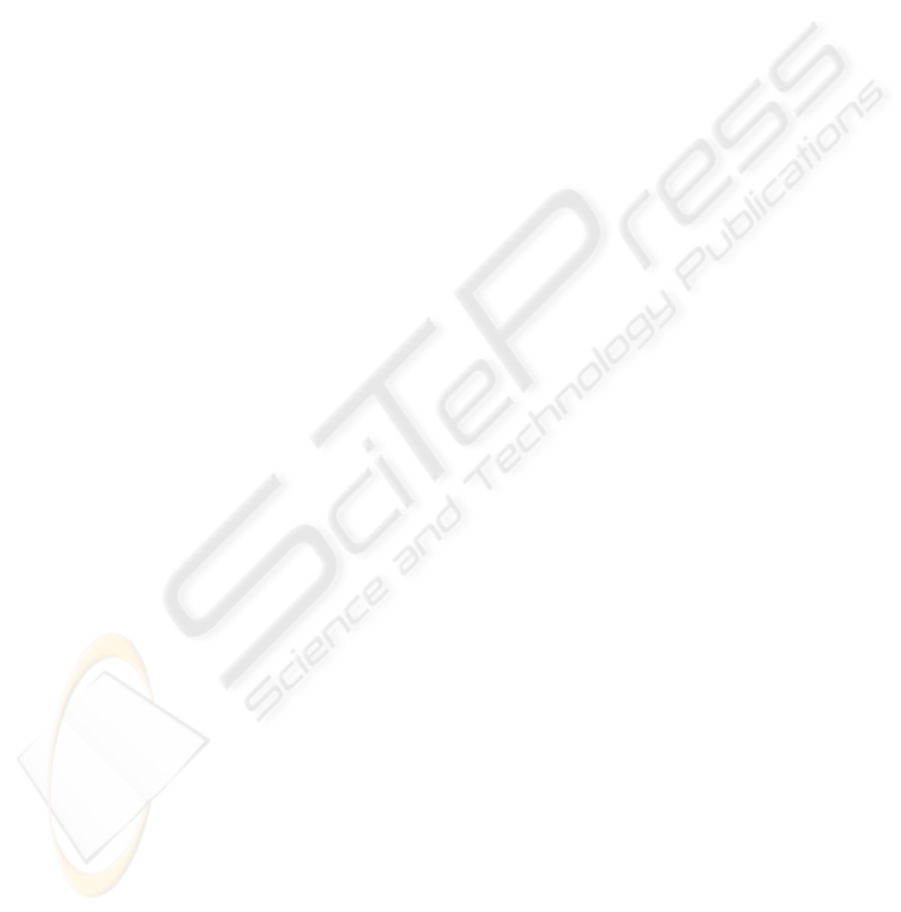
5 CONCLUSIONS
Examination of the puncture behaviour needles with
a silicone rubber model indicated that indentation
effects are significant at shallow insertion depths for
both silicone rubber and pig skin. Less than 50% of
the needle may be buried because of indentation
when short microneedles are used. The investigation
also revealed that the retraction force providing
mechanical stability to the needle in the skin was
linearly proportional to the buried depth and the
needle diameter. Short microneedles with small
diameters will have low retraction forces and poor
mechanical resistance against being dislodged.
ACKNOWLEDGEMENTS
This work was supported by grants from Hong
Kong’s Research Grants Council. The authors also
acknowledge the use of equipment provided by the
Advanced Engineering Materials facility at the
HKUST.
REFERENCES
Orive, G., Gascon, A.R., Hernandez, R.M., Dominguez-
Gil, A., Pedraz, J.L., 2004. Techniques: New
approaches to the delivery of biopharmaceuticals,
Pharma. Sci., Vol 25 No. 7 pp. 382-7.
Stoeber, B., Liepmann, D., 2005. Arrays of hollow out-of
plane microneedles for drug delivery, J. MEMS Vol
14 No. 3 pp. 474-9.
Staples, M., Daniel, K., Cima, M.J., Langer, R., 2006.
Application of micro- and nano-electromechanical
devices to drug delivery, Pharma Res. Vol 23 No. 5
pp. 847-63.
Ji, J., Tay, F.E.H., Miao, J., Iliescu, C., 2006.
Microfabricated microneedle with porous tip for drug
delivery, J. Micromech. Microeng. Vol 16 pp. 958-
964.
Nordquist, L., Roxhed, N., Griss, P., Stemme, G., 2007.
Novel microneedle patches for active insulin delivery
are efficient in maintaining glycaemic control: An
initial comparison with subcutaneous administration,
Phama. Res. (in print).
Parker, E.R., Rao, M.P., Turner, K.L., Meinhart, C. D.,
MacDonald, N.C., 2007. Bulk micromachined titanium
microneedles, J. MEMS Vol 16 No. 2 pp. 289-95.
Park, J., Allen, M.G., Prausnitz, M.R., 2006. Polymer
microneedles for controlled-released drug delivery,
Pharma. Res. Vol 23 No. 5 pp. 1008-19.
Davis, S. P., Wijaya, M., Allen, M. G., Prausnitz, M. R.,
2005. Hollow metal microneedles for insulin delivery
to diabetic rats, IEEE Trans. Biomed. Eng. Vol 52
No.5 pp. 909-15.
Sammoura, F., Kang, J.J., Heo, Y., Jung, T.S., Lin, L.,
2007. Polymeric microneedle fabrication using a
microinjection molding technique, Microsys. Tech..
vol 13 Issue 5 pp. 517-22.
Nguyen, C.T., Vu-Khanh, T., 2004. Mechanics and
mechanisms of puncture of elastomer membranes, J.
Mater. Sci. Vol 39 No.4 pp. 7361-4.
Sherwood O.A., Fleck, N.A., 2004. Mechanisms of deep
penetration of soft solids with application to the
injection and wounding of skin, Proc. R. Soc. Lon. A
vol. 460 pp. 3037-68.
Okamura, A. M., Simone, C., O’Leary, M. D., 2004.
Force Modeling for Needle Insertion Into Soft
Tissue,IEEE Trans. Biomed. Eng. Vol 51 No.10 pp.
1707-1716.
BIODEVICES 2008 - International Conference on Biomedical Electronics and Devices
296