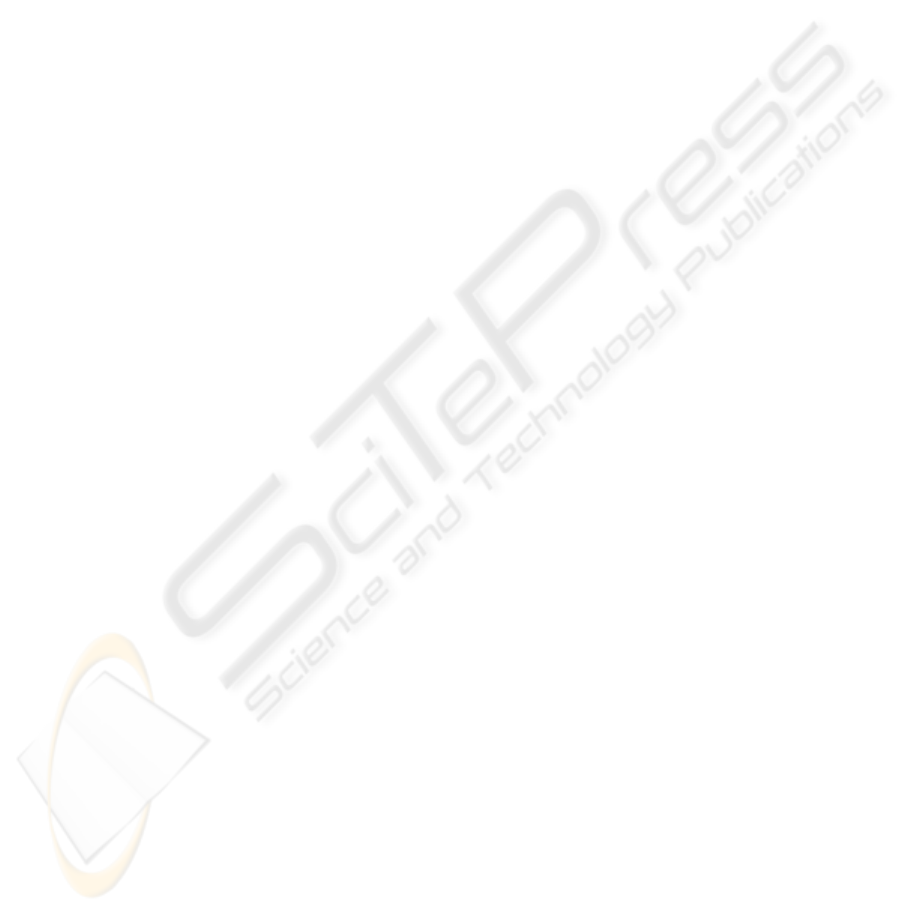
contribute to debilitating diseases such as
osteoporosis and osteopenia (Lane & Vigorita,
1983) and require additional treatment options.
However, controlled electrical stimulation and
mechanical loading may act as a synergistic catalyst
of bone ingrowth (Spadaro, 1997) and maintain host
bone bed integrity with elderly patients using an
osseointegrated electrical implant system.
Establishing tools for enhancing skeletal
attachment may assist with reducing the length of
rehabilitation required for an osseointegrated
procedure. Current programs require 2 to 24 months
of rehabilitation (Branemark, Branemark, Rydevik,
& Myers, 2001), a lengthy time period to ensure
uniform ingrowth. Because of the slow biological
process of skeletal attachment, loading at the
implant interface would be restricted for several
months after the operative procedure (Hofmann,
Bloebaum, & Bachus, 1997). However, using an
electrical stimulation system may enhance ingrowth
and allow patients to return to earlier ambulation.
4.1 Limitation
Since the conductivity of a titanium implant
significantly exceeded that of cortical bone, the
current densities at the implant interface should be
modeled to ensure localized tissue heating does not
occur, which may lead to patient discomfort or
potential tissue necrosis. Computational modeling of
current density fields are attainable with the given
software package and will be utilized in future work.
Additional efforts with be paid to altering the
porosity of the titanium implant and determining the
effect on the predicted electric fields since porosity
and conductivity are inversely related and may
affect the model as well (Ke et al., 2007).
5 CONCLUSIONS
The simulations developed for the proposed
biomedical device may have the capabilities of
expediting skeletal attachment by increasing
osteoblast migration. Computation modeling has
effectively shown that 1-10 V/cm electric fields may
be generated using the implant as a functional
cathode and topically applied anode band and
patches. Implementing computational models may
be the first step to resolving the classic problem with
electrical stimulation which is the inability to define
current pathways in the human body (Chakkalakal &
Johnson, 1981; Noda & Sato, 1985).
Patient specific modeling was effective for
attaining values that may be osteogenic at the
implant site, but wide variations in electric field
distributions shown in histograms reaffirm the need
to evaluate each case specifically. However, in order
to determine the accuracy of finite element analysis,
the quantity of subjects will be increased in the
future work to determine if an electrode
configuration could be optimized for patients with
percutaneous osseointegrated implants. Additional
model validation of electrically enhanced
osseointegration will be assessed using a small in
vivo animal model based on computational evidence
in future work.
ACKNOWLEDGEMENTS
This material is based upon research supported by
the Office of Research and Development,
Rehabilitation R&D Service, DVA SLC Health Care
System, Salt Lake City, Utah; the Albert & Margaret
Hofmann Chair and the Department of
Orthopaedics, University of Utah School of
Medicine, Salt Lake City, Utah. Additional technical
support for the simulations was provided by the
Center for Integrative Biomedical Computing of
Scientific Computing and Imaging Institute and was
made possible in part by software from the
NIH/NCRR Center for Integrative Biomedical
Computing, P41-RR12553-07.
REFERENCES
Agins, H. J., Alcock, N. W., Bansal, M., Salvati, E. A.,
Wilson, P. D., Pellicei, P. M., et al. (1988). Metallic
wear in failed titanium-alloy total hip replacements. J.
Bone Joint Surg. [Am.], 70-A(3), 347-356.
Albrektsson, T., Branemark, I.-I., Hansson, H.-A., &
Lindstrom, J. (1981). Osseointegrated titanium
implants. Acta Orthop Scand, 52, 155-170.
Albrektsson, T., & Albrektsson, B. (1987).
Osseointegration of bone implants. A review of an
alternative mode of fixation. Acta Orthop Scand,
58(5), 567-577.
Beder, O. E., & Eade, G. (1956). An investigation of
tissue tolerance to titanium metal implants in dogs.
Surgery, 39(3), 470-473.
Bloebaum, R. D., Bachus, K. N., Momberger, N. G., &
Hofmann, A. A. (1994). Mineral apposition rates of
human cancellous bone at the interface of porous
coated implants. J Biomed Mater Res, 28(5), 537-544.
BIODEVICES 2009 - International Conference on Biomedical Electronics and Devices
184