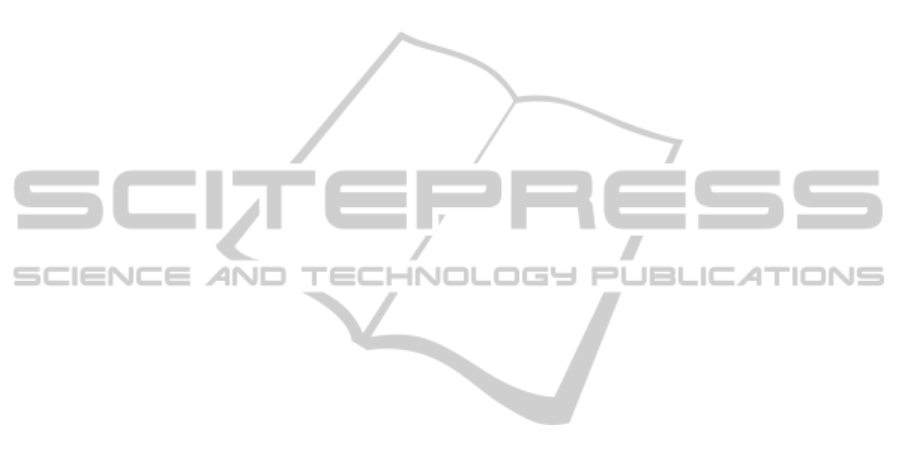
means to further improve articulatory stability during
recordings. We propose, at least, the following:
1. The test subject should be familiar with the MRI
noise as well as the cue signal so as to perform
optimally in the experimental situation.
2. The intensity of the cue signal should match the
MRI noise so that the initial voice production can
be maintained, i.e., possible sound intensity fluc-
tuation should be avoided.
3. The MRI noise itself is periodic and interferes
with the voice f
0
when they are close. Hence, the
cue should be matched with the MRI noise fre-
quency profile.
4. The voice sample f
0
should be standardized but in
a way that depends on the test subject.
5. The cue signal should be longer to allow the sub-
ject more time to inhale.
6. Externally triggered MRI sequences can be used
to introduce noiseless pauses.
All in all, the results from the current experiment are
encouraging. They clearly point to directions where
the setup refinements and better understanding will
iteratively approach a useful solution to the whole
problem.
REFERENCES
Aalto, A. (2009). A low-order glottis model with
nonturbulent flow and mechanically coupled acoustic
load. Master’s thesis, TKK, Helsinki. Available at
http://math.tkk.fi/research/sysnum/.
Aalto, A., Alku, P., & Malinen, J. (2009). A LF-pulse from
a simple glottal flow model. MAVEBA 2009 (pp. 199–
202). Florence, Italy.
Aalto, D., Aaltonen, O., Happonen, R., Malinen, J., Palo,
P., Saunavaara J., & Vainio, M. (2011). Recording
speech sound and articulation in MRI. Analysis and post-
processing of audio-spatial data. In preparation.
Branderud, P. (2008). Personal communication.
Bresch, E., Nielsen, J., Nayak, K., & Narayanan, S. (2006).
Synchronized and noise-robust audio recordings during
realtime magnetic resonance imaging scans (L). Jour-
nal of the Acoustical Society of America, 120(4), 1791 –
1794.
Dedouch, K., Hor´aˇcek, J., Vampola, T., &
ˇ
Cern´y, L. (2002).
Finite element modelling of a male vocal tract with con-
sideration of cleft palate. Forum Acusticum. Sevilla,
Spain.
Ericsdotter, C. (2005). Articulatory-Acoustic Relationships
in Swedish Vowel Sounds. PhD thesis, Stockholm Uni-
versity, Stockholm, Sweden.
Fant, G. (1960). Acoustic Theory of Speech Production.
Mouton, The Hague.
Hannukainen, A., Lukkari, T., Malinen, J., & Palo, P.
(2007). Vowel formants from the wave equation. Jour-
nal of the Acoustical Society of America Express Letters,
122(1), EL1–EL7.
Helmholtz, H. L. F. (1863). Die Lehre von den Tonempfind-
ungen als physiologische Grundlage fr dieTheorie der
Musik. Braunschweig: F. Vieweg.
Houde, J., & Jordan, M. (1998). Sensorimotor adaptation
in speech production. Science, 279(5354), 1213.
Kelso, J., Tuller, B., Vatikiotis-Bateson, E., & Fowler, C.
(1984). Functionally specific articulatory adaptation to
jaw perturbations during speech: Evidence for coordi-
native structures. Journal of Experimental Psychology,
10(6), 812–832.
Lu, C., Nakai, T., & Suzuki, H. (1993). Finite element sim-
ulation of sound transmission in vocal tract. J. Acoust.
Soc. Jpn. (E), 92, 2577 – 2585.
Lukkari, T., Malinen, J., & Palo, P. (2007). Recording
Speech During Magnetic Resonance Imaging. MAVEBA
2007 (pp. 163 – 166). Florence, Italy.
Malinen, J., & Palo, P. (2009). Recording speech during
MRI: Part II. MAVEBA 2009 (pp. 211–214). Florence,
Italy.
Mrayati, M., Carr, R., & Guerin, B. (1988). Distinctive
regions and modes: a new theory of speech production.
Speech Communication, (7), 257–286.
Niemi, M., Laaksonen, J., Peltomaki, T., Kurimo, J., Aalto-
nen, O., & Happonen, R. (2006). Acoustic comparison
of vowel sounds produced before and after orthognathic
surgery for mandibular advancement. Journal of Oral &
Maxillofacial Surgery, 64(6), 910–916.
Nishimoto, H., Akagi, M., Kitamura, T., & Suzuki, N.
(2004). Estimation of transfer function of vocal tract ex-
tracted from MRI data by FEM. The 18th International
Congress on Acoustics, Vol. II (pp. 1473 –1476). Kyoto,
Japan.
Vahatalo, K., Laaksonen, J., Tamminen, H., Aaltonen, O.,
& Happonen, R. (2005). Effects of genioglossal mus-
cle advancement on speech: an acoustic study of vowel
sounds. Otolaryngology - Head & Neck Surgery, 132(4),
636–640.
ˇ
Svancara, P., & Hor´aˇcek, J. (2006). Numerical Modelling of
Effect of Tonsillectomy on Production of Czech Vowels.
Acta Acustica united with Acustica, 92, 681 – 688.
ˇ
Svancara, P., Hor´aˇcek, J., & Peˇsek, L. (2004). Numerical
modelling of production of Czech Wovel /a/ based on FE
model of the vocal tract. Proceedings of International
Conference on Voice Physiology and Biomechanics.
RECORDING SPEECH SOUND AND ARTICULATION IN MRI
173