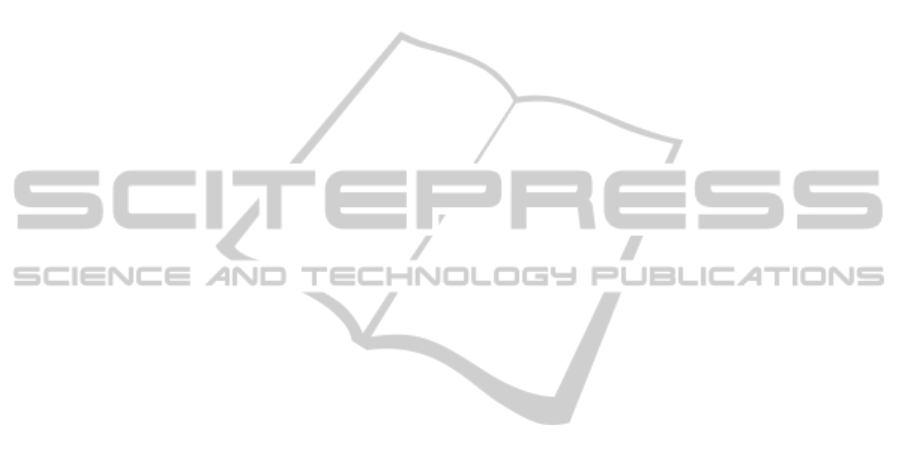
control of modelling and remodelling (Sorrentino et
al., 2007; Frost, 1990; Wolff, 1892; Frost, 1964;
Frost, 1994). Early studies by Wolff (1892) stated that
mechanics could determine changes in the
architecture of bones (Wolff, 1892). In 1964 Frost
expressed mathematically the reactions of the bone
tissue to given stimuli to quantitatively assess bone
deformations (Frost, 1964). Remodelling processes
repair the damage removing and replacing the
damaged tissues with new bone. Moreover,
overloading (or under-loading) alters such
phenomenon (Frost, 1994). Mechanically compatible
hydrogels as scaffolding materials could increase
prosthesis adaptation mechanisms introducing active
interfaces that improve implant biomimetics while
reproducing cartilage and ligaments bio-mechanical
functions. Adaptive properties of bone benefit of use
of biomimetic (biomechanically compatible and
bioactive) scaffold bio-materials.
2 MATERIALS AND METHODS
Our Biomimetic and Biomechanical approach
resulted from a parallel mechanical and physical
characterization of new hybrid material coupled to
the bio-mechanical Finite Element analysis of the
biological system investigated (implanted bones).
The mechanics of the “in vitro dynamic bender
testing apparatus” were designed by using FEA
analysis utilising the material properties of the
swollen hybrid pHEMA based nanocomposites.
2.1 Materials
Commercial 2-hydroxyethyl methacrylate, was
purchased from Sigma-Aldrich Chemicals Co., (St.
Louis, MO, USA). Fumed silicon dioxide (Aerosil
300 Degussa, Germany) with a mean diameter of 7
nm and specific surface area of 300 m
2
⋅g
−1
was
utilized as the bioactive filler. The initiator, α-α’
azoisobutyrronitrile (AIBN), was purchased from
Fluka (Milan, Italy).
HEMA monomers were mixed with increasing
amount of fumed silica (4 to 30% by volume),
according to the procedures described in a previous
work. The resin was poured in 10 mm diameter
cylindrical moulds, polymerized in a forced air
circulation oven set at 60°C for 24 hrs and finally
postcured at 90°C for 1 h.
2.2 Sorption and Swelling Test
The cylindrical samples were used for the water and
isotonic saline (0.15 M NaCl) water solution
sorption and swelling experiments. The solution
uptakes were determined at equilibrium by
gravimetric measurements in a 0.1 mg Mettler
Toledo balance (Milan, Italy). The advancing
swelling fronts in the anomalous Case II (Apicella
and Hopfenberg, 1982) of the samples were
monitored measuring the thickness of the un-swollen
residual glassy core as a function of time.
The equilibrium sorption and swelling experiments
were performed at 37°C (thermostatic water bath)
until constant weight up-take was monitored (100 h).
2.3 Finite Elements Analysis
Finite Element Analysis (FEA) on models of the
Titanium implanted bones (human mandible
segment) and of the in vitro bender set-up was
performed according to the following procedures.
2.3.1 Models Set-up
Implanted bone (human mandible section)
The solid models were generated using Solidwork
2007 software. Titanium implant and the
surrounding part of a mandibular cortical and
cancellous bone were modelled. The average
anatomical dimensions of the maxillary bone were
generated according to literature data (Schwartz-
Dabney, 2003) as a cancellous core surrounded by
2.0 mm-thick cortical bone. The FE model was
obtained by importing the solid models into ANSYS
rel. 9.0 FEM software (Ansys Inc. Houston) using
IGES format. The volumes were meshed with eight
nodes brick with 3 degree of freedom per node,
resulting in a 3D FE model made up of 31,240
elements and 35,841 nodes. The model was
constrained at the top surface of the maxillary bone.
Accuracy of the model was checked by convergence
tests (Sorrentino et al., 2007).
Piezoelectric Bender
The geometry of the piezoelectric bender has been
measured on the commercial product (see section 2.5)
and transferred to the FE environment according to
the procedures described for the implanted bone. A 3
mm thickness symmetrical layers of rubber hydrogel
were modelled at the two piezoelectric bender
surfaces (simulating a thick coating of our swollen
pHEMA based hybrid composite)
2.3.2 Mechanical Properties of Materials
Orthotropic assumption for cortical bone was
adopted while the cancellous bone was considered as
isotropic linear materials. The Young’s modulus and
A BIOMIMETIC AND BIOMECHANICAL APPROACH FOR TISSUE ENGINEERING - Hybrid Nanomaterials and a
Piezoelectric Tunable Bending Apparatus for Mechanically Stimulated Osteoblast Cells Growth
281