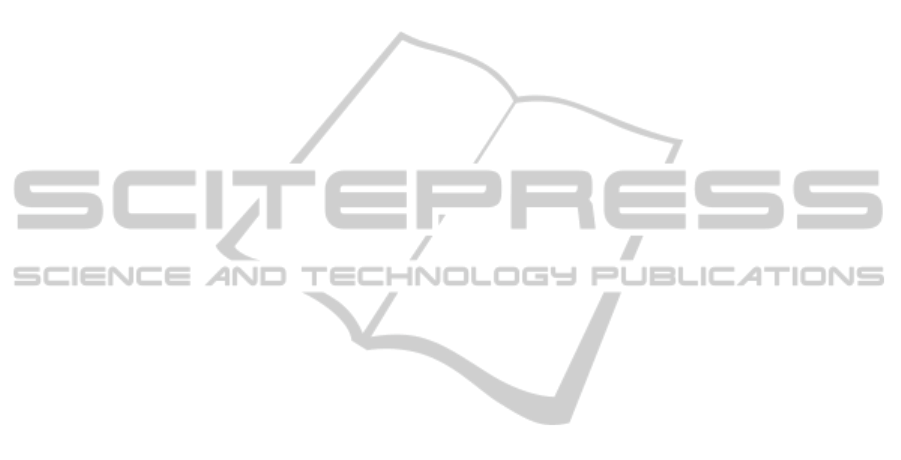
REFERENCES
Abrahamsen, A.B. et al., 2010. Superconducting wind
turbine generators. Superconductor Science and
Technology, 23(3), p.034019.
Ali, M.H., Minwon, P., et al., 2007. Improvement of wind
generator stability by fuzzy logic-controlled SMES.
Electrical Machines and Systems, 2007. ICEMS.
International Conference on, pp.1753-1758.
Ali, Mohd. Hasan, Murata, Toshiaki & Tamura, Junji,
2007. A Fuzzy Logic-Controlled Superconducting
Magnetic Energy Storage for Transient Stability
Augmentation. IEEE Transactions on Control Systems
Technology, 15(1), pp.144-150.
Arnold, G.W., 2011. Challenges and Opportunities in
Smart Grid: A Position Article. Proceedings of the
IEEE, 99(6), pp.922-927.
Arritt, R.F. & Dugan, R.C., 2011. Distribution System
Analysis and the Future Smart Grid. IEEE
Transactions on Industry Applications, 47(6),
pp.2343-2350.
Asao, T. et al., 2007. Smoothing control of wind power
generator output by superconducting magnetic energy
storage system. In Electrical Machines and Systems,
2007. ICEMS. International Conference on. pp. 302-
307.
Baumann, P.D., 1992. Energy conservation and
environmental benefits that may be realized from
superconducting magnetic energy storage. IEEE
Transactions on Energy Conversion, 7(2), pp.253-259.
Benysek, G., 2007. Improvement in the Quality of
Delivery of Electrical Energy using Power Electronics
Systems. 1 Edition ed. s.l.:Springer.
Buckles, W. & Hassenzahl, W.V., 2000. Superconducting
magnetic energy storage. IEEE Power Engineering
Review, 20(5), pp.16-20.
Cherian, S. & Ambrosio, R., 2004. Towards realizing the
gridwise~ vision: integrating the operations and
behavior of dispersed energy devices, consumers, and
markets. In IEEE PES Power Systems Conference and
Exposition. IEEE, pp. 38-43.
Comission, E., 2005. Towards Smart Power Networks -
Lessons learned from European research FP5
projects. s.l.:Office for Official Publications of the
European Communities.
Comission, E., n.d. Smart Grids European Technology
Platform. [Online] Available at:
http://www.smartgrids.eu/ [Accessed 6 January 2012].
EPRI, n.d. EPRI IntelliGrid. [Online] Available at:
http://www.intelligrid.epri.com/ [Accessed 6 January
2012].
Fanning, R. & Huber, R., 2005. Distribution vision 2010:
planning for automation. In IEEE Power Engineering
Society General Meeting. IEEE, pp. 2969-2970.
Farhangi, H., 2010. The path of the smart grid. IEEE
Power and Energy Magazine, 8(1), pp.18-28.
Ferrier, M., 1970. Stockage d'energie dans un enrolement
supraconducteur. Low Temperature and Electric
Power
, pp. 425-432.
Garrity, T., 2008. Getting Smart. IEEE Power and Energy
Magazine, 6(2), pp.38-45.
Grijalva, S. & Tariq, M.U., 2011. Prosumer-based smart
grid architecture enables a flat, sustainable electricity
industry. In ISGT 2011. IEEE, pp. 1-6.
Gungor, V. et al., 2011. Smart Grid Technologies:
Communications Technologies and Standards. IEEE
Transactions on Industrial Informatics, 7(4), pp.529-
539.
Hanson, D.J. et al., 2002. A STATCOM-based relocatable
SVC project in the UK for National Grid. In 2002
IEEE Power Engineering Society Winter Meeting.
Conference Proceedings. IEEE, pp. 532-537.
Hassenzahl, W.V., 2001. Superconductivity, an enabling
technology for 21st century power systems? IEEE
Transactions on Appiled Superconductivity, 11(1),
pp.1447-1453.
Hassenzahl, W.V. et al., 2004. Electric power applications
of superconductivity. Proceedings of the IEEE,
92(10), pp.1655-1674.
Hatziargyriou, N. et al., 2007. Microgrids. IEEE Power
and Energy Magazine, 5(4), pp.78-94.
Hsu, C.-S. & Lee, W.-J., 1993. Superconducting magnetic
energy storage for power system applications. IEEE
Transactions on Industry Applications, 29(5), pp.990-
996.
Hutson, C., Venayagamoorthy, G.K. & Corzine, K.A.,
2008. Intelligent Scheduling of Hybrid and Electric
Vehicle Storage Capacity in a Parking Lot for Profit
Maximization in Grid Power Transactions. In 2008
IEEE Energy 2030 Conference. IEEE, pp. 1-8.
Ipakchi, A. & Albuyeh, F., 2009. Grid of the future. IEEE
Power and Energy Magazine, 7(2), pp.52-62.
Jiang, S., Annakkage, U.D. & Gole, A.M., 2006. A
Platform for Validation of FACTS Models. IEEE
Transactions on Power Delivery, 21(1), pp.484-491.
Juengst, K.-P., 2002. Application studies of
superconducting fault current limiters in electric power
systems. IEEE Transactions on Appiled
Superconductivity, 12(1), pp.900-903.
Kadurek, P., Cobben, J.F.G. & Kling, W. L., 2010. Smart
MV/LV transformer for future grids. In SPEEDAM
2010. IEEE, pp. 1700-1705.
Kadurek, Petr, Cobben, J.F.G. & Kling, W. L., 2011.
Smart transformer for mitigation of voltage
fluctuations in MV networks. In 2011 10th
International Conference on Environment and
Electrical Engineering. IEEE, pp. 1-4.
Khan, U.A. et al., 2011. Feasibility Analysis of the
Positioning of Superconducting Fault Current Limiters
for the Smart Grid Application Using Simulink and
SimPowerSystem. IEEE Transactions on Applied
Superconductivity, 21(3), pp.2165-2169.
Kinjo, T. et al., 2006. Terminal-voltage and output-power
regulation of wind-turbine generator by series and
parallel compensation using SMES. IEE Proceedings -
Generation, Transmission and Distribution
, 153(3),
p.276.
Kovalsky, L. et al., 2005. Applications of Superconducting
Fault Current Limiters in Electric Power Transmission
SUPERCONDUCTINGMAGNETICENERGYSTORAGE-ATechnologicalContributetoSmartGridConcept
Implementation
119