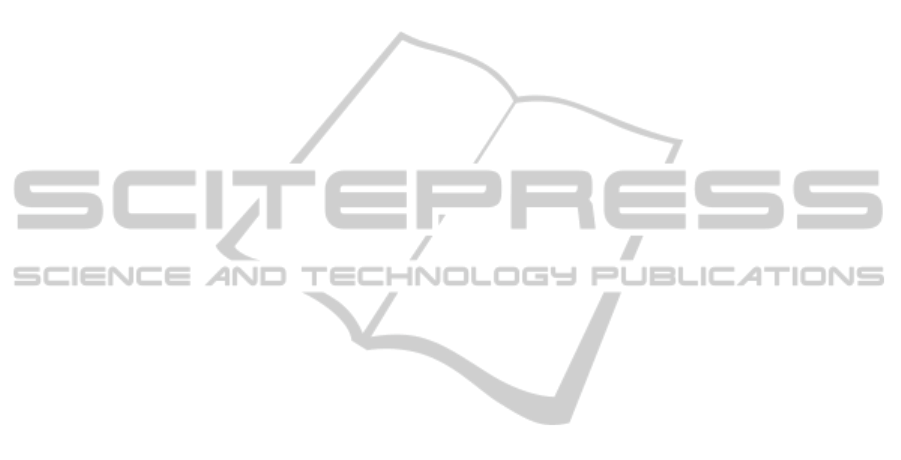
REFERENCES
D. A. B. Miller, 2000. “Optical Interconnects to Silicon,”
IEEE J. Sel. Top. Quant. 6, 1312-1317
S. Lecomte, R. Paschotta, S. Pawlik, B. Schmidt, K.
Furusawa, A. Malinowski, D.J. Richardson, U. Keller,
2005,“Optical parametric oscillator with a pulse
repetition rate of 39 GHz and 2.1-W signal average
output power in the spectral region near 1.5 um,” Opt.
Lett. 30, 290-292.
K. J. Weingarten, M.J.W. Rodwell, D.M. Bloom, 1988,
“Picosecond optical sampling of GaAs integrated
circuits,” IEEE J. Quantum Elect. 24, 198 -220.
A. Bartels, T. Dekorsy, H. Kurz, 1999 “Femtosecond
Tisapphire ring laser with a 2-GHz repetition rate and
its application in time-resolved spectroscopy,” Opt.
Lett. 24, 996-998.
R. Ramaswami, K. Sivarajan, 1998, Optical Networks A
Practical Perspective, San Mateo CA, Morgan
Kaufmann Publishers.
S. Hoogland, S. Dhanjal, A. C. Tropper, J. S. Roberts, R.
Häring, R. Paschotta, F. Morier-Genoud, U. Keller,
2000, “Passively Mode-Locked Diode-Pumped
Surface- Emitting Semiconductor Laser,” IEEE
Photonic. Tech. L. 12, 1135-1137.
R. Häring, R. Paschotta, A. Aschwanden, E. Gini, F.
Morier-Genoud, U. Keller, 2002, “ High-power
passively mode-locked semiconductor lasers, ” IEEE
J. Quantum Elect. 38, 1268-1275.
A. Aschwanden, D. Lorenser, H.J. Unold, R. Paschotta, E.
Gini, U. Keller, 2005, “10 GHz passively mode-locked
external-cavity semiconductor laser with 1.4W
average output power,” Appl. Phys. Lett. 86, 131102.
U. Keller, A. C. Tropper, 2006, “Passively modelocked
surface-emitting semiconductor lasers,” Phys. Rep.
429, 67-120.
T. Gherman, D. Romanini, I. Sagnes, A. Garnache, Z.
Zhang, 2004, “Cavity-enhanced absorption
spectroscopy with a mode-locked diode-pumped
vertical external-cavity surface-emitting laser,” Chem.
Phys. Lett. 390, 290-295.
P. Dupriez, C. Finot, A. Malinowski, J. K. Sahu, J.
Nilsson, D. J. Richardson, K. G. Wilcox, H. D.
Foreman, A. C. Tropper, 2006, “High-power, high
repetition rate picosecond and femtosecond sources
based on Yb-doped fiber amplification of VECSELs,”
Opt. Express 14, 9611-9616.
Z. Mihoubi, K. G. Wilcox, S. Elsmere, A. Quarterman, R.
Rungsawang, I. Farrer, H. E. Beere, D. A. Ritchie, A.
C. Tropper, V. Apostolopoulos, 2008, “All -
semiconductor room -temperature terahertz time
domain,” Opt. Lett. 33, 2125-2127.
P. Klopp, U. Griebner, M. Zorn, A. Klehr, A. Liero, M.
Weyers, G. Erbert, 2009, “ Mode-locked InGaAs-
AlGaAs disk laser generating sub-200fs pulse,” Opt.
Express 17, 1820-1834.
D. Lorenser, D. J. H. C. Maas, H. J. Unold, A. R.
Bellancourt, B. Rudin, E. Gini, D. Ebling, U. Keller,
2006, “50-GHz Passively Mode-Locked Surface-
Emitting Semiconductor Laser With 100-mW Average
Output Power,” IEEE J. Quantum Elect. 42, 838-847
A. Aschwanden, D. Lorenser, H. J. Unold, R. Paschotta,
E. Gini, U. Keller, 2005, “ 2.1-W Picosecond
Passively Mode-Locked External-Cavity
Semiconductor Laser,” Opt. Lett. 30, 272-274.
A. C. Tropper, H. D. Foreman, A. Garnache, K. G.
Wilcox, S. H. Hoogland, 2004, “Vertical-external-
cavity semiconductor lasers,” J. Phys. D: Appl. Phys.
37, R75-R85.
R. Paschotta, R. Haring, A. Garnache, S. Hoogland, A. C.
Tropper, U. Keller, 2002, “Soliton-Like Pulse-Shaping
Mechanism in Passively Mode-Locked Surface-
Emitting Semiconductor Lasers,” Appl. Phys. B-
Lasers O. 75, 445-451.
OPTICS2012-InternationalConferenceonOpticalCommunicationSystems
364