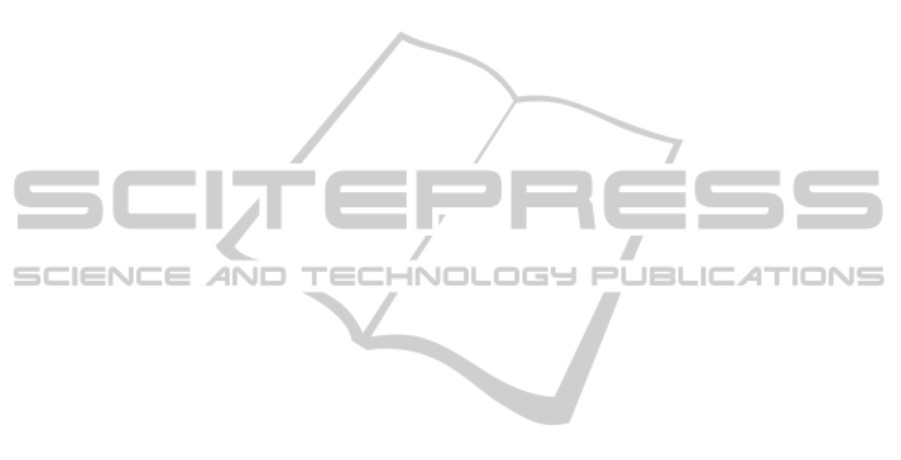
SV. Consequently, the mass and protein packing in
SV is maximal.
5 CONCEPTUAL REMARKS
We postulate that variants in N1-TAIL are attractive
to cope with changing metabolic and activity status
of the synapse.
We suggest that in addition to the regulation for
uORFs (upstream ORFs) and activation of
alternative splicing, the translation regulation is an
additional mode for fine-tuning the overall protein
production. Investigating translational signals along
the transcripts of synapse is only in its infancy. We
expect methods such as ribosomal profiling (Ingolia,
et al., 2009) to provide quantitative data for
translation speed and efficiency.
An open question that we began to explore
challenges the translational management with
respect to ribosomal subunits in dendrites (and other
neuronal compartment) (Sutton and Schuman,
2006). We postulate that local translation is critical
for fast and efficient translation under conditions of
restricted resources. Recently, it was shown that
hundreds of mRNAs are localized to neuronal
compartments such as synaptic neuropils (Cajigas, et
al., 2012). Translation of such mRNA must be
highly regulated at the levels of transcript
accessibility and the translation efficiency. Here, we
provide a glimpse on an overlooked evolutionary
encoded signal for managing translation of synaptic
proteins.
ACKNOWLEDGEMENTS
We thank Amos Stern for useful discussions. The
work is supported by Prospects EU FRVII
consortium.
REFERENCES
Ames, A., 3rd (1992) Energy requirements of CNS cells as
related to their function and to their vulnerability to
ischemia: a commentary based on studies on retina,
Can J Physiol Pharmacol, 70 Suppl, S158-164.
Arava, Y., et al. (2003) Genome-wide analysis of mRNA
translation profiles in Saccharomyces cerevisiae, Proc
Natl Acad Sci U S A, 100, 3889-3894.
Barrell, D., et al. (2009) The GOA database in 2009-an
integrated Gene Ontology Annotation resource,
Nucleic Acids Res, 37, D396-403.
Brachya, G., et al. (2006) Synaptic proteins as multi-
sensor devices of neurotransmission, BMC Neurosci,
7 Suppl 1, S4.
Broadie, K.S. and Richmond, J.E. (2002) Establishing and
sculpting the synapse in Drosophila and C. elegans,
Curr Opin Neurobiol, 12, 491-498.
Cajigas, I.J., et al. (2012) The local transcriptome in the
synaptic neuropil revealed by deep sequencing and
high-resolution imaging, Neuron, 74, 453-466.
Chiti, F. and Dobson, C.M. (2006) Protein misfolding,
functional amyloid, and human disease, Annu Rev
Biochem, 75, 333-366.
dos Reis, M., et al. (2004) Solving the riddle of codon
usage preferences: a test for translational selection,
Nucleic Acids Res, 32, 5036-5044.
Ferro-Novick, S. and Jahn, R. (1994) Vesicle fusion from
yeast to man, Nature, 370, 191-193.
Gebauer, F. and Hentze, M.W. (2004) Molecular
mechanisms of translational control, Nat Rev Mol Cell
Biol, 5, 827-835.
Gingold, H. and Pilpel, Y. (2011) Determinants of
translation efficiency and accuracy, Mol Syst Biol, 7,
481.
Holcik, M., et al. (2000) Internal ribosome initiation of
translation and the control of cell death, Trends Genet,
16, 469-473.
Ikemura, T. (1985) Codon usage and tRNA content in
unicellular and multicellular organisms, Mol Biol
Evol, 2, 13-34.
Ingolia, N.T., et al. (2009) Genome-wide analysis in vivo
of translation with nucleotide resolution using
ribosome profiling, Science, 324, 218-223.
Lucks, J.B., et al. (2008) Genome landscapes and
bacteriophage codon usage, PLoS Comput Biol, 4,
e1000001.
Mahlab, S., et al. (2012) Conservation of the relative
tRNA composition in healthy and cancerous tissues,
RNA. 18, 640-652.
Marais, G. and Duret, L. (2001) Synonymous codon
usage, accuracy of translation, and gene length in
Caenorhabditis elegans, J Mol Evol, 52, 275-280.
Martin, K.C., et al. (2000) Local protein synthesis and its
role in synapse-specific plasticity, Curr Opin
Neurobiol, 10, 587-592.
Mutch, S.A., et al. (2011) Protein quantification at the
single vesicle level reveals that a subset of synaptic
vesicle proteins are trafficked with high precision, J
Neurosci, 31, 1461-1470.
Nestler, E.J. (2001) Molecular basis of long-term plasticity
underlying addiction, Nat Rev Neurosci, 2, 119-128.
Percudani, R. (2001) Restricted wobble rules for
eukaryotic genomes, Trends Genet, 17, 133-135.
Pielot, R., et al. (2012) SynProt: A Database for Proteins
of Detergent-Resistant Synaptic Protein Preparations,
Front Synaptic Neurosci, 4, 1.
Plotkin, J.B. and Kudla, G. (2010) Synonymous but not
the same: the causes and consequences of codon bias,
Nat Rev Genet, 12, 32-42.
Richter, J.D. and Sonenberg, N. (2005) Regulation of cap-
dependent translation by eIF4E inhibitory proteins,
BIOINFORMATICS2013-InternationalConferenceonBioinformaticsModels,MethodsandAlgorithms
156