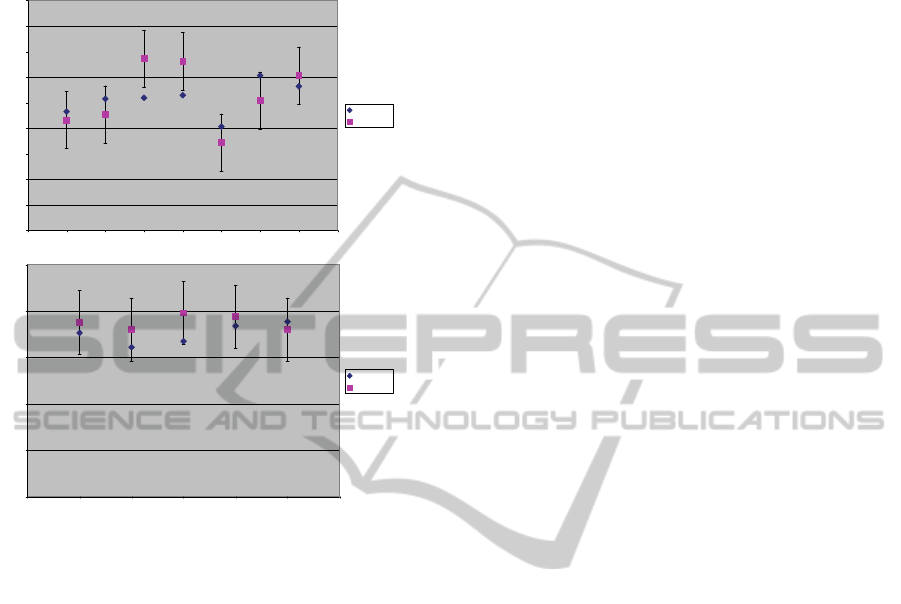
device and other methods, verifying the potential of
the device to measure lung function parameters
noninvasively.
0
0.5
1
1.5
2
2.5
3
3.5
4
4.5
012345678
(a) Male Volunteer
Lung Volume
Measured
Expected
0
0.5
1
1.5
2
2.5
0123456
b
Female Volunteer
Lung Volume
Measured
Expected
Figure 4: Functional residual capacity estimated by the
device for healthy simultaneously-breathing subjects.
4 FUTURE RESEARCH
We will continue to carry more studies of the device
in the clinical environment such as besides the
bedside and in the intensive care unit. We are
currently obtaining ethnic approval to test the device
on diseased patients. Patients who come in the lung
function for pulmonary test will be asked to
volunteer 30 mins to be tested by our device. The
test results will then be compared to other tests such
as body plethysmography, spirometry, and impulse
oscillometry (which are already gathered through the
patients’ normal test procedure). We hope to gather
more evidence of the effectiveness of the device
through this study.
We will further refine the design and miniaturize
the device, making it more suitable for the clinical
environment. In particular, we aim to make the
device weighted less than 30kg, mounted on a 40cm
x 30 cm x 100cm trolley which can be moved easily
around the crowded intensive care environment.
Our goal is to complete an advanced prototype,
which is readily marketable to medical device
manufacturers by 2014.
REFERENCES
Farmery, A. D. & Hahn, C. E. W., 2001. A method of
reconstruction of clinical gas-analyzer signals
corrupted by positive-pressure ventilation. Journal of
Applied Physiology, 90(4), pp.1282–1290.
Farmery, A. D. & Hahn, C. E. W., 2000. Response-time
enhancement of a clinical gas analyzer facilitates
measurement of breath-by-breath gas exchange.
Journal of Applied Physiology, 89(2), pp.581–589.
Gavaghan, D. J. & Hahn, C. E. W., 1996. A tidal breathing
model of the forced inspired inert gas sinewave
technique. Respiration Physiology, 106(2), pp.209–
221.
Hahn, C. E. et al., 1993. Gas exchange in a three-
compartment lung model analyzed by forcing
sinusoids of N2O. Journal of Applied Physiology,
75(4), pp.1863–1876.
Hahn, C. E., 1996. Oxygen respiratory gas analysis by
sine-wave measurement: a theoretical model. Journal
of Applied Physiology, 81(2), pp.985–997.
Ibañez, J. & Raurich, J. M., 1982. Normal values of
functional residual capacity in the sitting and supine
positions. Intensive Care Medicine, 8(4), pp.173–177.
King, G. G., 2011. Cutting edge technologies in
respiratory research: Lung function testing.
Respirology, 16(6), pp.883–890.
Whiteley, J. P., Gavaghan, D. J. & Hahn, C. E. W., 2000.
A tidal breathing model of the inert gas sinewave
technique for inhomogeneous lungs. Respiration
Physiology, 124(1), pp.65–83.
Williams, E. M. et al., 1997. Alveolar and Dead Space
Volume Measured by Oscillations of Inspired Oxygen
in Awake Adults. Am. J. Respir. Crit. Care Med.,
156(6), pp.1834–1839.
Williams, E. M. et al., 1994. Assessment of
cardiorespiratory function using oscillating inert gas
forcing signals. Journal of Applied Physiology, 76(5),
pp.2130–2139.
Williams, E. M. et al., 1996. Measurement of respiratory
parameters by using inspired oxygen sinusoidal
forcing signals. Journal of Applied Physiology, 81(2),
pp.998–1006.
Williams, E. M. et al., 1998. Pulmonary blood flow
measured by inspiratory inert gas concentration
forcing oscillations. Respiration Physiology, 113(1),
pp.47–56.
Zwart, A., Seagrave, R. C. & Dieren, A. V., 1976.
Ventilation-perfusion ratio obtained by a noninvasive
frequency response technique. Journal of Applied
Physiology, 41(3), pp.419–424.
Zwart, Aart et al., 1978. A non-invasive determination of
lung perfusion compared with the direct Fick method.
Pflügers Archiv European Journal of Physiology,
375(2), pp.213–217.
BIODEVICES2013-InternationalConferenceonBiomedicalElectronicsandDevices
152