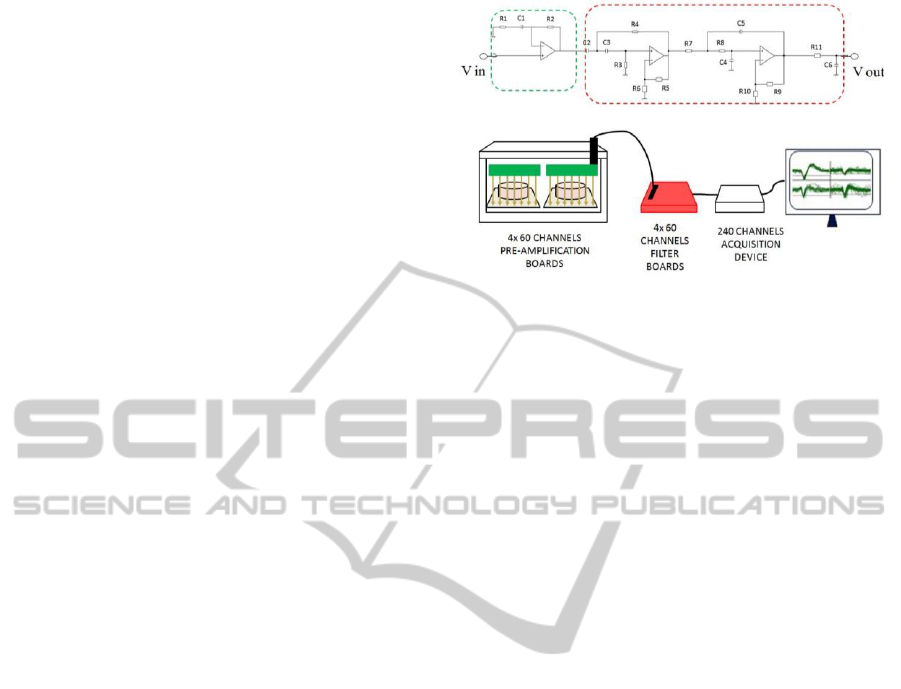
humidification. A Nickel-Chrome heating wire (10
W), insulated by a silicon sheath, is placed into the
bottle to worm up the gas flow. This element is
powered on when a miniaturized humidity sensor
(SHT15, Sensirion AG), integrated into the chamber,
measures values of relative humidity (RH) lower
than 85% and it is switched off when RH reaches
95%. In its first version, the control is implemented
by software (USB6009 and Labview, National
Instruments). An heating resistive wire is bound to
the tube which connects the bubbling module to the
chamber, to prevent air cooling. To have a reference
for the miniaturized sensor, RH measurements after
the bubbling column and inside the environmental
chamber have been performed with a commercial
probe (HMP233, Vaisala Inc.).
A high RH level could induce condensation in
the chamber, thus raising the probability of water
droplets to drain on the cells and decreasing
visibility from outside. Condensation of the water
vapour over the inner face of the top plate would
arise if its temperature lowers under the dew point.
To avoid this drawback, heating elements could be
placed on the top plate. To suitably design the
heaters, a FEM model of the steady-state heat
transfer through the whole environmental chamber
has been implemented (Comsol).
2.2 MEA Recording Boards Design
To perform electrical recordings from cultures
grown on four 60-channel MEA chips, custom pre-
processing boards have been designed and coupled
to a multichannel commercial data acquisition
system (USB-ME240, 50 kHz, 16 bit, Multi Channel
Systems, MCS, GmbH).
The whole processing chain was designed to
fulfil the main following requirements: (1) high gain
(~ 60 dB), suitable for the amplification of neuronal
signals in vitro (20-400 μV peak to-peak ) (2)
neuronal spike bandwidth (300Hz - 5 kHz), (3) low
noise (< 20 μV peak-to-peak, i.e., typical thermal
and background biological noise at the
microelectrodes). The defined circuitry (Figure 2,
top) consists of a pre-amplification stage (gain 100,
high pass cut frequency at 300 Hz), a Butterworth
high-pass filter (gain 3, cut frequency at 300 Hz) and
a Butterworth low pass filter followed by a RC low
pass (gain 3, cut frequency at 5 kHz). The
performance of the circuit was simulated with Spice
software (LTSpice, Linear Technology).
Figure 2: Schematic of the custom front-end circuitry (top)
and assembly of the boards (bottom).
For each 60 channel MEA, the pre-amplification
stage was designed to be implemented on two 30-
channel boards (65x65 mm) placed inside the culture
chamber (Figure 2, in green), in order to avoid the
degradation of the signal-to-noise ratio (SNR) of
recordings. A squared hole has been drilled in the
centre of each board in order to allow visibility of
the cultures and to insert tubes from medium
exchange from the top. The following filter stage
was implemented on two 100x100 mm 30-channel
external boards (Figure 2, in red). Surface mounted
components and low noise, precision opamps were
chosen. Four copies of pre-amplifiers and filter
modules were realized in order to record
simultaneously from four MEAs (i.e., 240 channels).
The assessment of the real frequency response
gain of each board was obtained by providing sine
waves (peak-to-peak amplitude equal to 100 μV for
the pre-amplification stage and 10 mV for the filter
stage) by means of a sinusoidal wave generator with
frequency varying between 1 Hz and 10 kHz, for
each channel. The input-referred noise (300 Hz - 5
kHz) of each board was tested measuring the
channel output and dividing it by the overall
nominal gain, with inputs connected to ground. The
channel crosstalk was measured by sending a 1 kHz
controlled sine wave to one channel, and recording
from directly adjacent ones (with inputs grounded).
After testing each board independently, pre-
amplification and filter boards were connected and
tested together.
2.3 Electrophysiological Recordings
From Neuronal Cultures
An Experimental Platform Aimed at Long Lasting Electrophysiological Multichannel Recordings of Neuronal Cultures
553