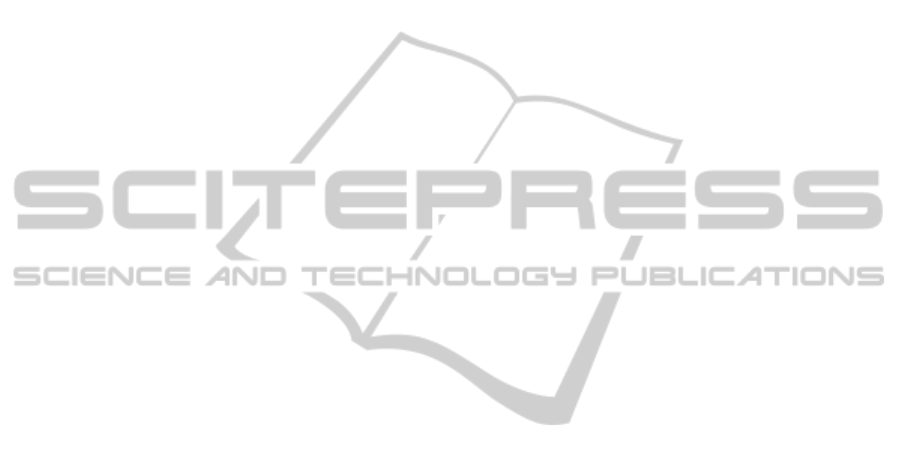
medium, which is an important parameter and is not
insignificant for the treatment of RL.
The lower photodegradation period was
associated to the higher chemical stability provided
by the silica shell, which should establish a potential
barrier for the charge transfer.
ACKNOWLEDGEMENTS
We gratefully acknowledge financial support from
Rede 36 Nanobiotec CAPES (Brazil). V.M. thanks
the CAPES (Brazil) for doctoral fellowships.
REFERENCES
Lawandy, N. M., Balachandran, R. M., Gomes, A. S. L. &
Sauvain, E., 1994. Nature, 368, 436–438.
Polson R. C., Chipoline A. and Vardeny Z. V., 2001.
Advanced Materials, 13, 760-764.
Masaru Sakai, Yuta Inose, Kazuhiro Ema, Tomi Ohtsuki,
Hiroto Sekiguchi, Akihiko Kikuchi, and Katsumi
Kishino, 2010. Appl. Phys. Lett., 97, 151109.
M. N. Shkunov, M. C. DeLong, M. E. Raikh, Z. V.
Vardeny, A. A. Zakhidov and R. H. Baughman,
Synthetic Metals, 2001, 116, 485-491.
Wiersma D. and Cavalier S., 2001. Nature, 414, 708-709.
Schuurmans, F. J. P., Vanmaekelbergh, D., van de
Lagemaat, J. & Lagendijk, A. 1999. Science, 284,
141–143.
Cao H., Ling Y., Xu J. Y., Cao C. Q. and Kumar P., 2001.
Phys. Rev. Lett., 86, 4524-4527.
Tianrui Zhai, Xinping Zhang, Zhaoguang Pang, Xueqiong
Su, Hongmei Liu, Shengfei Feng, and Li Wang, 2011.
Nano Lett., 11 (10), 4295–4298.
Noginov M. A., 2005. “Solid-State Random Lasers,”
(Springer Series in Optical Sciences), Norfolk State
University, Norfolk.
Hui Cao, 2005. J. Phys. A: Math. Gen., 38, 10497–10535.
Wiersma D. S., 2008. Nature Physics, 4, 359-367.
Sha W., Liu C.-H., and Alfano R., 1994. J.Opt. Soc.
Amer., B 19, 1922–1924.
Noginov M. A., Caulfield H. J., Noginova N. E., and
Venkateswarlu P., 1995. Opt. Commun., 118, 430–
437.
Leonetti M., Conti C. and Cefe Lopez, 2012. Appl. Phys.
Lett., 101, 051104.
Mandzy N., Grulke E., Druffel T., 2005. Powder
Technology, 160, 121–126.
Sabzi M., Mirabedini S.M., Zohuriaan-Mehr J., Atai M.,
2009. Progress in Organic Coatings, 65, 222–228.
Brito-Silva A. M., André Galembeck, Anderson S. L.
Gomes, Alcenisio Jesus-Silva J., Cid B. de Araújo,
2010. Journal of Applied Physics, 108, 033508.
Joseph N. Ryan , Menachem Elimelech , Jenny L.
Baeseman , Robin D. Magelky, 2000. Environ. Sci.
Technol., 34, 2000-2005.
E. Jimenez-Villar, Valdeci Mestre, Paulo C. de Oliveira,
Gilberto F. de Sá, 2013. Nanoscale, in press;
DOI:10.1039/C3NR03603K.
B-E. Jimenez-Villar, Valdeci Mestre, Paulo C. de Oliveira,
Wagner M. Faustino, D. S. Silva, Gilberto F. de Sá,
2013, Appl. Phys, Lett., Submitted.
Fox M. A., Dulay M. T., 1993. Chem. Rev., 93, 341.
E. Jimenez, Kamal Abderrafi, Juan Martınez-Pastor,
Rafael Abargues, Jose Luıs Valdes, Rafael Ibañez,
2008. Superlattices and Microstructures, 43, 487–493.
Ernesto Jimenez, Kamal Abderrafi, Rafael Abargues, Jose
L. Valdes, Juan P. Martınez-Pastor, 2010. Langmuir,
26 (10), 7458–7463.
Gustavo Fuertes, Orlando L. Sanchez-Munoz, Esteban
Pedrueza, Kamal Abderrafi, Jesus Salgado, Ernesto
Jimenez, 2011. Langmuir, 27, 2826–2833.
B-Gustavo Fuertes, Esteban Pedrueza, Kamal Abderrafi,
Rafael Abargues, Orlando Sánchez, Juan Martínez-
Pastor, Jesús Salgado, Ernesto Jiménez, 2011. Proc.
SPIE Medical Laser Applications and Laser-Tissue
Interactions V, No. 8092-51.
Rodriguez E., Jimenez E., Jacob G. J., Neves A. A. R.,
Cesar C. L., Barbosa
L. C., 2005. Physica E, 26, 361.
Rodriquez E., Kellerman G., Jiménez E., César C. L.,
Barbosa L. C., 2008. Superlattices and
Microstructures, 43 (5-6), 626-634.
Stöber W., Fink A., and Bohn E., 1968. J. Colloid
Interface Sci.,26, 62.
Sheng-Li Chen, Peng Dong, Guang-Hua Yang, 1997.
Journal of Colloid and Interface Sci., 189 (2), 268–
272.
Abderrafi Kamal, Jimenez Ernesto, Ben T., Molina S. I.,
Ibáñez R., Chirvony V., Martínez-Pastor J. P., 2012. J.
Nanoscience and Nanotechnology, 12 (8), 6774-6778.
Basudeb Karmakar, Goutam De, Dibyendu Ganguli, 2000.
Journal of Non-Crystalline Solids, 272, 119–126.
Gijs van Soest and Ad Lagendijk, 2002. Physical Review
E, 65, 047601.
Noginov M. A., Caulfield H. J., Noginova N. E.,
Venkateswarlu P., 1995. Optics Communications, 118,
430-437.
Yang Zhang, Yongsheng Chen, Paul Westerhoff, Kiril
Hristovski, John C Crittenden, 2008. Water Research,
42 (8-9), 2204–2212.
Chih-ping Tso, Cheng-min Zhung, Yang-hsin Shih,
Young-Ming Tseng, Shian-chee Wu and Ruey-an
Doong, 2010. Water Science & Technology, 61 (1),
127-133.
van G., Wold A., 1993. Chem. Mater., 5, 280.
Amy L. Linsebigler, Guangquan Lu, and John T. Yates,
1995. Jr. Chem. Rev., 95, 735-758.
Serpone, N., Pelizzetti, E., 1989. “Photocatalysis-
Fundamentals and Applications”, Eds.; Wiley: New
York Chapter 7, van Damme, H. Supports in
Photocatalysis.
Tsukamoto M., Abe N., Soga Y., Yoshida M., Nakano H.,
Fujita M., Akedo J., 2008. Appl Phys A, 93, 193–196.
HighEfficiencyandLowPhotodegradationinRandomLaser,usingNovelTiO2@SilicaNanoparticles
31