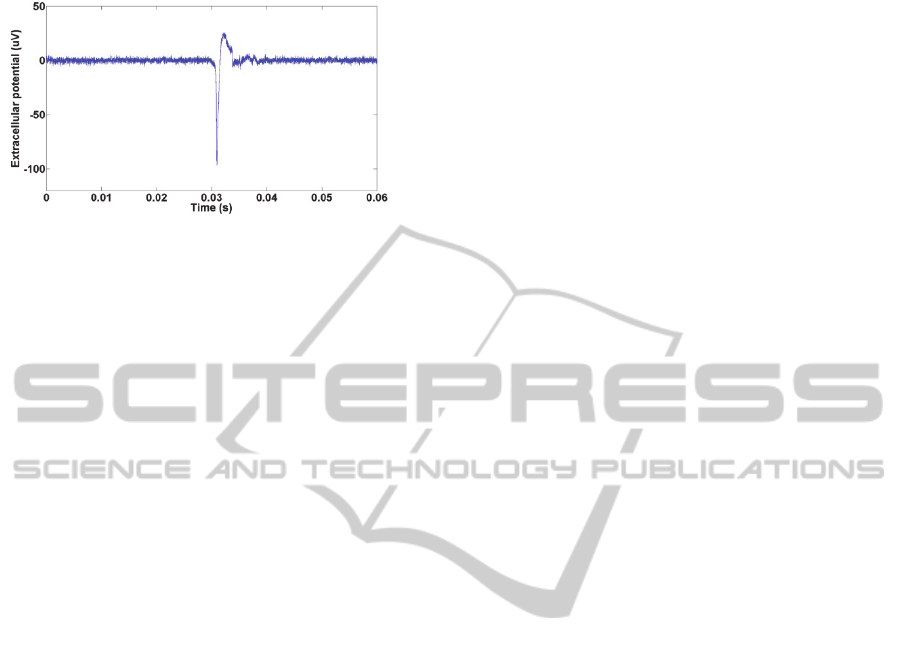
neurons on the MEA was further verified.
Figure 6: A spontaneous neuronal spike recorded from
electrode 11 in Figure 5.
4 CONCLUSIONS
Dielectrophoresis is used, with increasing frequency,
in combination with microdevices, to manipulate
biological cells. However, it is important to
understand the impact the implementation of DEP
may have on the viability of cells. In this work we
have investigated the viability of mouse
hippocampal neurons positioned on the electrodes of
microfabricated multi-electrode arrays after the
implementation of pDEP. We showed that neurons
maintained high viability after short-term exposure
to cell-trapping solution, which contained, primarily,
10% sucrose. With electric signal of appropriate
frequency and amplitude (such as 6Vpp and 10MHz),
neuron membrane breakdown was prevented during
the DEP process. Most importantly, we have
obtained electrical signals from the neurons
positioned on the MEA, 12 hours after using positive
dielectrophoresis, further confirming the health and
electrically active properties of neurons.
ACKNOWLEDGEMENTS
This work was partially funded by National Science
Foundation (NSF) grant NSF ECCS-1321356 and a
grant to Lehigh University from the Howard Hughes
Medical Institute (HHMI) through the Precollege
and Undergraduate Science Education Program.
REFERENCES
Berdondini, L., Imfeld, K., Maccione, A., Tedesco, M.,
Neukom, S., Koudelka-Hep, M., and Martinoia, S.,
2009. Active pixel sensor array for high spatio-
temporal resolution electrophysiological recordings
from single cell to large scale neuronal networks. Lab
Chip, 9, 2644–2651.
Buzsaki, G., Anastassiou, C. A., and Koch, C., 2012. The
origin of extracellular fields and currents – EEG,
ECoG, LFP and spikes. Nat. Rev. Neurosci., 13, 407-
420.
Chitwood, R. A., Hubbard, A., and Jaffe, D. B., 1999.
Passive electrotonic properties of rat hippocampal
CA3 interneurones. J. Physiol., 515(3), 743-756.
Gagnon, Z. R., 2011. Cellular dielectrophoresis:
applications to the characterization, manipulation,
separation and patterning of cells. Electrophoresis, 32,
2466-2487.
Gentet, L. J., Stuart, G. J., and Clements, J. D., 2000.
Direct measurement of specific membrane capacitance
in neurons. Biophys. J., 79, 314-320.
Gupta, V., Jafferji, I., Garza, M., Melnikova, V. O.,
Hasegawa, D. K., Pethig, R., and Davis, D. W., 2012.
ApoStream, a new dielectrophoretic device for
antibody independent isolation and recovery of viable
cancer cells from blood. Biomicrofluidics, 6, 024133.
Heida, T., Vulto, P., Rutten, W. L. C., and Marani, E.,
2001. Viability of dielectrophoretically trapped neural
cortical cells in culture. J. Neurosci. Methods, 110, 37-
44.
Heida, T., Wagenaar, J. B., Rutten, W. L. C., and Marani,
E., 2002. Investigating membrane breakdown of
neuronal cells exposed to nonuniform electric fields by
finite-element modeling and experiments. IEEE Trans.
Biomed. Eng., 49(10), 1195-1203.
Ho, C. T. et al., 2013. Liver-cell patterning lab chip:
mimicking the morphology of liver lobule tissue. Lab
Chip, 13, 3578-3587.
Hochberg, L. R. et al., 2006. Neuronal ensemble control of
prosthetic devices by a human with tetraplegia. Nat.,
442, 164–171.
Honegger, T., Scott, M. A., Yanik, M. F., and Voldman,
J., 2013. Electrokinetic confinement of axonal growth
for dynamically configurable neural networks. Lab
Chip, 13, 589-598.
Huang, C., Liu, C., Loo, J., Stakenborg, T., and Lagae, L.,
2014. Single cell viability observation in cell
dielectrophoretic trapping on a microchip. Appl. Phys.
Lett., 104, 013703.
Jaber, F. T., Labeed, F. H., and Hughes, M. P., 2009.
Action potential recording from dielectrophoretically
positioned neurons inside micro-wells of a planar
microelectrode array. J. Neurosci. Methods, 182, 225-
235.
Jeng, C. P., Huang, C. T., and Shih, H. Y., 2010.
Hydrodynamic separation of cells utilizing insulator-
based dielectrophoresis. Microsystem Technologies,
16(7), 1097-1104.
Jones, T. B., 1995. Electromechanics of Particles,
Cambridge University Press. New York, pp. 34-81.
LaLonde, A., Romero-Creel, M. F., and Lapizco-Encinas,
B. H., 2014. Assessment of cell viability after
manipulation with insulator-based dielectrophoresis.
Electrophoresis, 35, 1-6.
BIODEVICES2015-InternationalConferenceonBiomedicalElectronicsandDevices
76