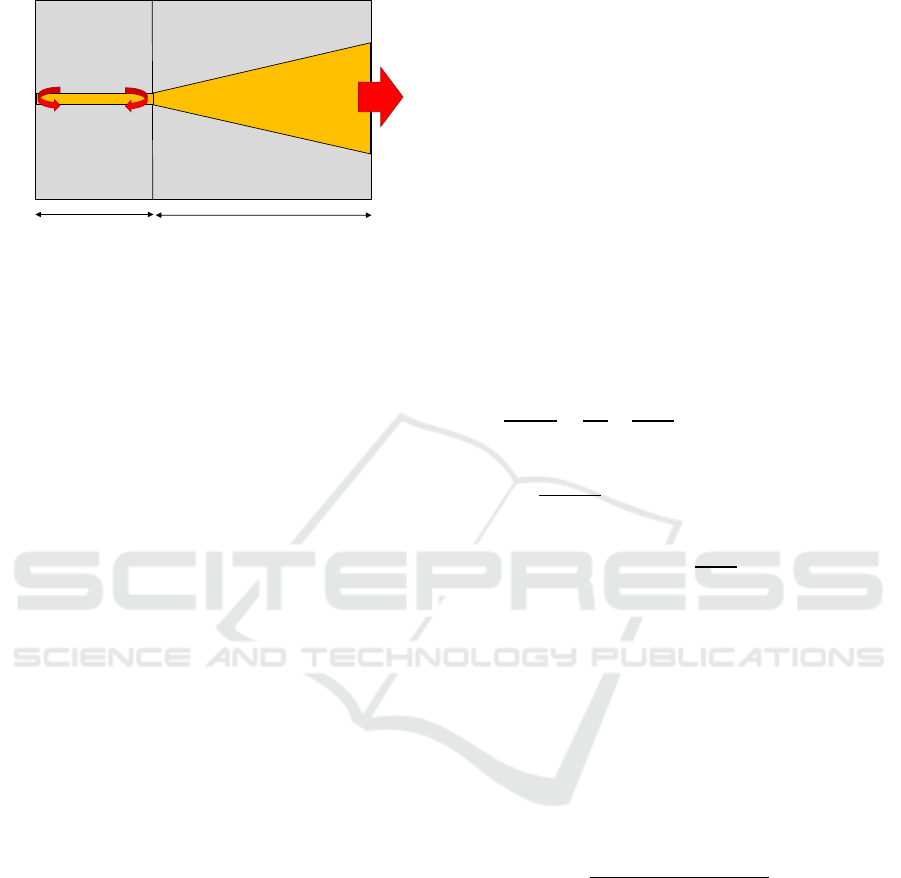
Figure 1: Schematic diagram of a single spatial mode
tapered master oscillator (MO) power amplifier (PA) laser.
In real tapered lasers, this ability to scale power while
maintaining single-mode performance is limited by
the stability of the lateral optical mode. The onset of
beam filamentation occurs in regions where the gain
is not well-saturated, such as along the edges of the
optical mode where the intensity is lower. Reflection
at the front facet creates a backwards-travelling wave
that can seed laser oscillation, especially in the
regions where the carrier density is high due to poor
gain clamping.
Because tapered amplifiers are edge-emitting
devices, typical measurement techniques do not
permit observation of the gain profile (though the
integrated amplified spontaneous emission can be
measured). In larger laser systems (solid state, fiber,
and gas lasers, for example), the gain can be measured
through the observation of the spontaneous emission
from the side of the laser cavity. High power tapered
semiconductor lasers, however, are bonded junction
down, and the excess solder that pools along the edge
of the device prevents investigation of the gain from
the side of the device. Thus, there exists a need for a
technique which allows for measurement of the gain
profile within the tapered amplifier, and the
demonstration of any such approach would greatly
benefit the development of tapered laser diodes.
In this paper, a method which permits direct
observation of the two-dimensional spontaneous
emission profile, and hence gain profile, within a
tapered optical amplifier is demonstrated. In the next
section, a model which accounts for coupling of the
photon density to the gain profile is developed. The
subsequent sections report the design of the device
and experimental results of the approach.
2 THEORY
2.1 Model Development
Modeling of the 2D photon and carrier distribution in
the tapered power amplifier was based on the
semiconductor laser rate equations and leveraged our
groups’ prior work modeling the effects of
longitudinal spatial hole burning in broad area
semiconductor lasers (Hao, 2014). The standard
semiconductor rate equations are modified to permit
variation of the local carrier density N(z), local
photon density
, and local gain
with
longitudinal position, as described in equations (1)
through (3) In a perfect optical amplifier, feedback
from the emitting facet (and cavity resonance) is
suppressed, thus it is only necessary to track the
forward propagating photon density.
The following parameters are defined as follows.
is the internal quantum efficiency, is the injection
current, and is the electron charge. is the volume
of the active region,
is the photons’ group
velocity, is the optical mode confinement factor,
is the intrinsic optical loss,
is the gain coefficient,
and
is the transparency carrier density. is the
carrier lifetime which includes Auger recombination,
Shockley-Read-Hall recombination, and spontaneous
radiative recombination (Chuang, 1995).
The finite difference method was used to solve the
simultaneous differential equations along the length
of the cavity. The length of the cavity was divided
into 50 steps and once the photon density was known
for the first point, it was calculated for the second, and
so on. This propagation process was repeated for
many steps in the width of the cavity so that a two-
dimensional model could be created.
Coupling of the photon population to the carrier
population results in an inverse relationship between
gain and photon density. Thus, as the injected signal
from the master oscillator is amplified along the z-
direction of the amplifier, gain and carrier density are
L
MO
L
PA
Single-Mode
Master Oscillator Tapered Power Amplifier
Direct Observation of the 2D Gain Profile in High Power Tapered Semiconductor Optical Amplifiers
115