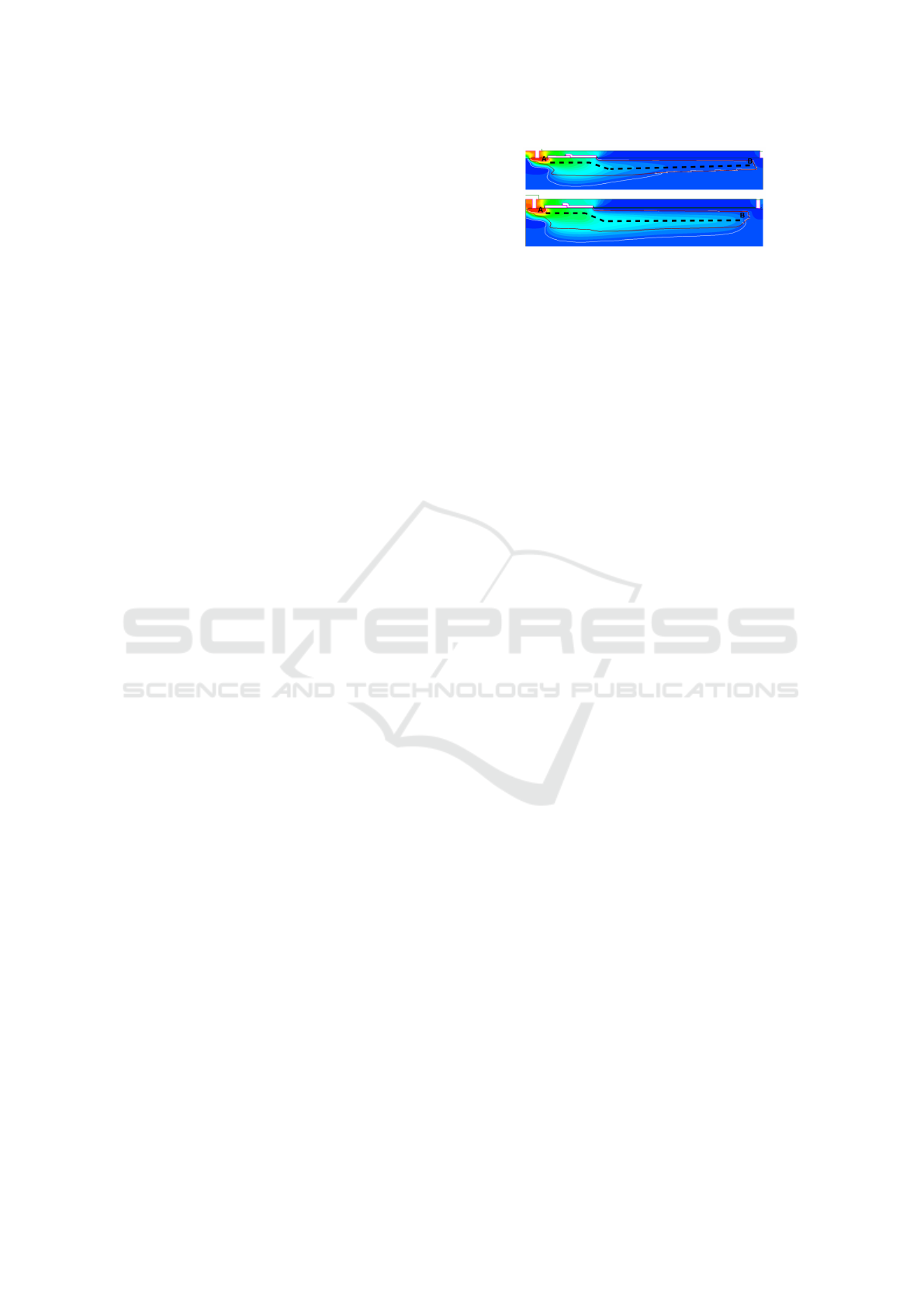
a built-in electrical field and the connection of the n-
well to the FD gives the opportunity to use the reset
voltage to implement a stronger electric field to the
diode as will be shown.
2 SIMULATION
The potential inside the n-well is pinned to a con-
stant value determined by the p+ top layer and the
p substrate and it depends on the doping concentra-
tions. Therefore, the dominating transport mecha-
nism is diffusion which is up to two orders of mag-
nitude slower than the electron transport in an electric
field. The pinned photodiode can be interpreted as a
simple p-n-p structure and the pinning voltge can be
calculated from the built-in potentials of the two p-n-
junctions. Thus the pinning voltage depends only on
the technology parameters, which are defined by the
implantation dose and implantation energy. There-
fore, the lateral doping gradient changes the pinning
voltage across the length of the diode and provides
a built-in electrical field. Since a gradient depends
on the length it is applied on, this effect vanishes in
bigger sensors. All previous mentioned solutions cre-
ate an internal field and are not implementable for
larger sensors (>15 µm). The key to increase the read-
out speed for large pixels is to introduce a potential
difference across the diode bigger than the pinning
voltage, which is only possible by bypassing the pin-
ning behaviour. We succeeded to achieve this with a
second well implantation to create a designated elec-
tron path with a slightly higher doping concentration.
The voltage distribution inside the n-well with and
without the second implantation is shown in figure
2. The readout voltage is not anymore suppressed by
the pinning behaviour. Also the depletion zone is ex-
panded. The implantation is applied at the end of the
front end of line so the thermal diffusion is negligi-
ble and the doping concentration increases only in a
distinct area of the well as an overall higher doping
concentration would reduce the width of the deple-
tion zone. However, the transition from the slightly
higher doping concentration of the n-well to the dop-
ing concentration of the readout node could result in
a barrier which slows down the electrons. Figure 3
shows the simulated conduction band energy across
the diode for different n-well implantation doses. The
barrier-free electron transport requires the conduction
band energy to follow a monotonic decreasing func-
tion. Figure 4 shows the maximum energy gradi-
ent under the CG as well as the maximum voltage
drop across the diode for the different implantation
doses. Without the second implantation the voltage
Figure 2: Simulation result of the voltage distribution across
the photodiode without the second implantation (top) and
with the second implantation (bottom). The electrons travel
through the diode on the path from B to A.
drop does not exceed 1 V. The simulations suggest to
set the target implantation dose to 3.0e11 cm
−2
. The
increased depletion region increases the quantum ef-
ficiency in the IR spectrum but decreases the well ca-
pacitance. The pixel performance is not affected by
the smaller capacitance as the electrons are stored in
the FD. Furthermore, a higher doping concentration
reduces the electron drift velocity ((Jacoboni et al.,
1977)) in a constant electrical field provided by the
pinning voltage. The decrease in mobility is compen-
sated by the 1.7 times stronger voltage gradient which
leads to an increased electron speed. Particularly the
strong electric field on the well interfaces drives the
electrons towards the readout node and the electron
velocity at these interfaces with the second implan-
tation is nearly two orders of magnitude higher. The
path-time-diagram for one electron based on the ve-
locity calculation (in figure 5) shows that the second
implantation can enhance the transfer time of the pho-
todiode doesn’t show a strong quadratic dependence
anymore. The influence of the strong field at the well
interface can be seen as the curve saturates. However,
these are the calculations for one electron and the time
to ensure complete charge transfer may be a factor of
5-10x longer in any PPD ((Fossum and Hondongwa,
2014)) especially if a barrier is present. It is important
for 3D-ToF measurements to reduce the transfer time
to a minimum as any remaining charge affects the dis-
tance calculation and thus reducing the measurement
range. A complete charge transfer after 10 ns is de-
sirable. Therefore, a diode length of 26 µm with the
second implantation could provide good results.
3 MEASUREMENT
We analyzed five different pixel configurations which
differ from each other by their length and implanta-
tion scheme. Table 1 shows the main differences be-
tween the measured devices. We extended the length
of the diode for the second device in order to see the
influence as the transfer time rises. The velocity simu-
lations are based on the implantation scheme of pixel
C. The higher doping concentration of 3.8e11 cm
−2
Optimization of Transfer Times in Pinned Photodiodes
313