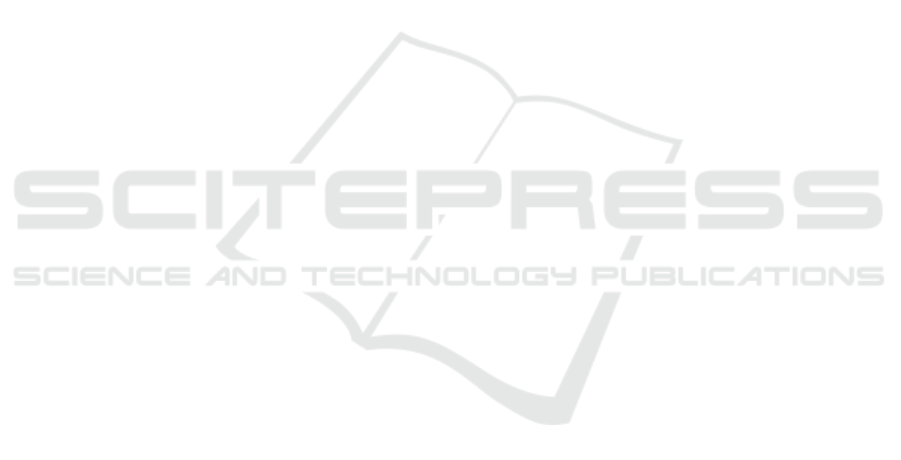
MEYS, and Czech Republic under the project
CANAM OP, CZ.02.1.01/0.0/0.0/16_013/0001812.
REFERENCES
Aithal, P. Sreeramana, Nagaraja, H. S., Mohan Rao, P,
Avasthi, D. K., and Sarma, Asati, Journal of Applied
Physics 81, 7526 (1997); doi: 10.1063/1.365294
Bányász, I., Fried, M., Dücső, Cs., and Vértesy, Z., Appl.
Phys. Lett., 79, 3755 (2001)
Bányász, I., S. Berneschi, M. Bettinelli, M. Brenci, et al.,
“MeV energy N
+
- implanted planar optical waveguides
in Er-doped tungsten-tellurite glass operating at 1.55
µm”, IEEE Photonics Journal, Volume 4, Issue 3, pp.
721-7, DOI: 10.1109/JPHOT.2012.2194997 (2012)
Bányász, I., Z. Zolnai, M. Fried, T. Lohner, et al., “Single -
and double energy N
+
ion irradiated planar optical
waveguides in Er: Tungsten-Tellurite Oxide glass and
sillenite type Bismuth Germanate crystals for telecom
applications”, Nuclear Instruments and Methods in
Physics Research Section B, 307, 299-304 (2013)
Berneschi, S., G. Nunzi Conti, I. Bányász, et al., “Ion beam
irradiated channel waveguides in Er
3+
-doped tellurite
glass", Applied Physics Letters, 90, 121136, (2007)
Berneschi, S, Brenci, M., Nunzi Conti, G., Pelli, S., et al.,
Slab optical waveguides in Er
3+
-doped tellurite glass by
N
+
ion implantation at 1.5 MeV, Optical Engineering,
50, 071110 (2011).
Chen, F.,
Wang, Xue-Lin and Wang, Ke-Ming, Opt. Mat.,
29, 1523-1542, DOI: 10.1016/j.optmat.2006.08.001
(2007).
Dong, N., Jaque, D., Chen, F. and Lu, Q., “Second
harmonic and Raman imaging of He
+
implanted
KTiOPO4 waveguides”, Optics Express, 19, 13934-
13939 (2011).
Gibbons, J.F., Proceedings of the IEEE, 60, 1062 – 1096,
(1972), DOI: 10.1109/PROC.1972.8854
He, Ruiyun, Shuqian Sun, Miaomiao Xu, Feng Chen,
Shavkat Akhmadaliev, Shengqiang Zhou, Nucl. Instr.
and Meth. in Phys. Res. B, 308, 6-8 (2013)
Li, S.- L. Han, P., Shi, M., Yao, Y., et al., “Low-loss
channel optical waveguide fabrication in Nd
3+
-doped
silicate glasses by femtosecond laser direct writing”,
Optics Express, 19, 23958-23964 (2011).
Liu Chun-Xiao; Xu Jun; Xu Xiao-Li; Wu Shu; Wei Wei;
Guo Hai-Tao; Li Wei-Nan; and Peng Bo, Optical
Engineering, 53(3), 037101 (2014)
Jaouen, Y., du Mouza, L., Barbier, D. Delavaux, J., Bruno,
P., “Eight-wavelength Er-Yb doped amplifier:
combiner/splitter planar integrated module”, IEEE
Photonics Technology Letters, 11, 1105 – 1107 (1999).
Miller, S. E. “Integrated Optics: An introduction”, Bell
System Technical Journal, 48, 2059 – 2069 (1969).
Ogoshi, H., Ichino, S. and Kurotori, K., “Broadband Optical
Amplifiers for DWDM Systems”, Furukawa Review,
No. 19, 17-21 (2000).
Ohishi, Y., Mori, A., Yamada, M., Ono, H., et al., “Gain
characteristics of tellurite-based erbium-doped fiber
amplifiers for 1.5 μm broadband amplification”, Optics
Letters, 23, 274-276 (1998).
Olivares, J., García, G., Agulló-López, F., Agulló-Rueda,
F., Kling, A., Soares, J.C., Appl. Phys. A, 81, 1465–
1469 (2005) DOI: 10.1007/s00339-005-3237-x
Olivares, J., García, García-Navarro, Agulló-López, F., A.,
Caballero, O., García-Cabañes, A, Appl. Phys. Lett., 86,
183501 (2005)
Opfermann, Th., Höche, Klaumünzer, S., Wesch, W, Nucl.
Instr. and Meth. in Phys. Res. B, 166-167, 954-958
(2000)
Peña-Rodríguez, O., Olivares, J., Carrascosa, M., García-
Cabañes, A., Rivera A. and Agulló-López, F., “Optical
Waveguides Fabricated by Ion Implantations/Ir-
radiation: A Review”, in: Ion Implantation, Prof. Mark
Goorsky (Ed.), ISBN: 978-953-51-0634-0, InTech,
Available from: http://www.intechopen.com/books/
ion-implantations/optical-waveguides-fabricated-by-
ion-implantation-irradiation-a-review (2012)
Ren, Y., Tan, Y., Chen, F., Jaque, D., et al, “Optical channel
waveguides in Nd: LGS laser crystals produced by
proton implantation”, Optics Express, 18, 16258-16263
(2010).
Sohler, W., Das, B., Dey, D., Reza, S., et al., “Erbium-
Doped Lithium Niobate Waveguide Lasers”, IEICE
Trans. Electron., E88-C, 990-997 (2005).
Tan Y, Chen F, Physica Status Solidi-Rapid Research
Letters 1 (6), 277-279 (2007)
Townsend, P. D., Chandler, P. J.
and Zhang, L., Optical
Effects of Ion Implantation, Cambridge University
Press, Cambridge, U.K. (1994)
Xu, Kaikai, Liu, Haitao and Zhang, Zhengyuan, “Gate-
controlled diode structure based electro-optical
interfaces in standard silicon-CMOS integrated
circuitry”, Applied Optics, 54, 6420-6424 (2015)
Yao, Y., Tan, Y., Dong, N., Chen, F. and Bettiol, A. A.,
“Continuous wave Nd: YAG channel waveguide laser
produced by focused proton beam writing”, Optics
Express, 18, 24516-24521 (2011).
Yao, Y., Dong, N., Chen, Vanga, S. K.
and Bettiol, A. A.,
“Proton beam writing of Nd: GGG crystals as new
waveguide laser sources”, Optics Letters, 36 4173-4175
(2011).
Wooten, E. L.
et al., “A Review of Lithium Niobate
Modulators for Fiber-Optic Communications
Systems”, IEEE Journal of Selected Topics in Quantum
Electronics, 6, 69-82 (2000).
Zhao, Jin-Hua, Liu, Xiu-Hong, Huang, Qing, Liu, Peng,
Wang Lei and Wang, Xue-Lin, Nuclear Instruments
and Methods in Physics Research Section B: Beam
Interactions with Materials and Atoms, 268, 2923–
2925 (2010)
Ziegler, J.F., “SRIM-2003”, Nucl. Instr. and Meth.B, 219–
220, 1027 (2004), and http://www.srim.org
Zou, J., Zhao, F. and Chen, R. T., “Two-step K
+
-Na
+
and
Ag
+
-Na
+
ion-exchanged glass waveguides for C-band
applications”, Applied Optics, 41, 7620-7626 (2002).
Design, Ion Beam Fabrication and Test of Integrated Optical Elements
285