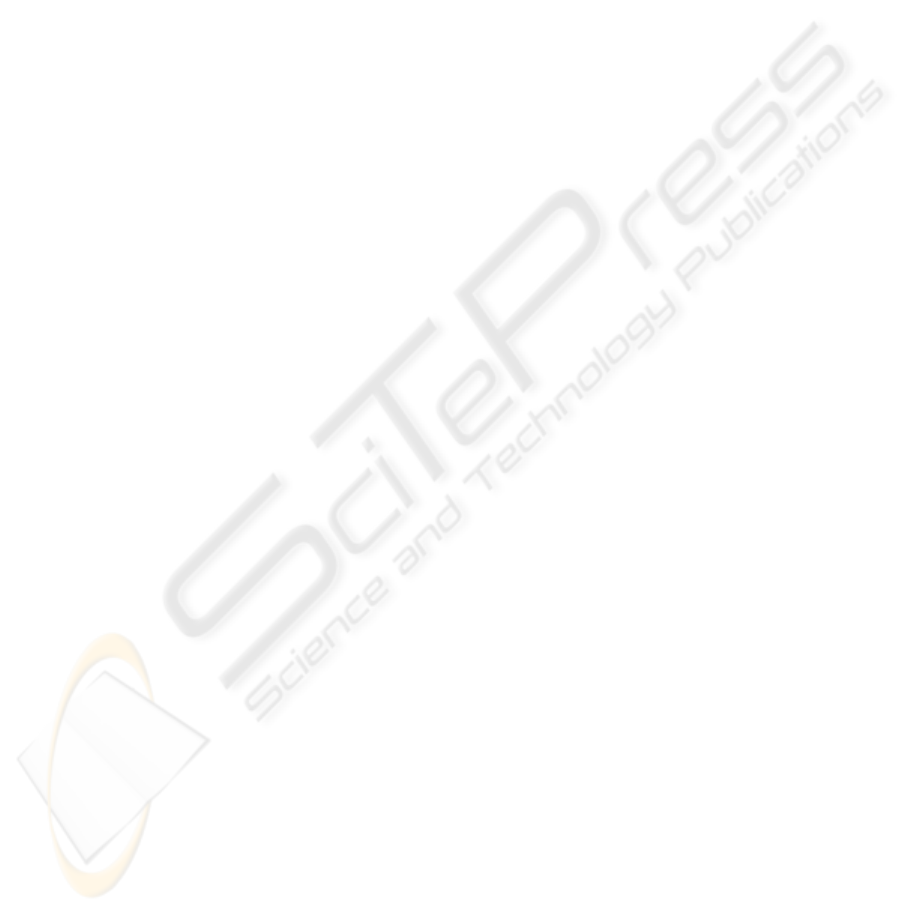
devices of the multisensor layer were shown and
their mechanical characteristics discussed,
particularly, it demonstrated excellent flexibility for
good skin conformity. It was also demonstrated that
information on bodily motion due to cardiac
contraction, or BCG signals, can be acquired
through sensors integrated on the proposed platform.
The system further shows potential for medical
grade diagnostic performance. Further testing and
characterization of more compact and highly-
integrated models of the proposed system is under
development, and will ultimately provide more
insightful understanding of the effectiveness of the
proposed microintegration platform.
ACKNOWLEDGEMENTS
The authors would like to thank Jasbir Patel from the
Computational Integrative BioEngineering Research
Lab and Microfluidics Lab at Simon Fraser
University for his help on silicone microfabrication.
The authors would also like to thank See-Ho Tsang
from the Institute for Micromachining and
Microfabrication Research at Simon Fraser
University for his help on polyimide circuit
fabrication. Further, the authors would like to
acknowledge CMC Microsystems for their ongoing
support in hybrid micro integration and device
fabrication assistance.
REFERENCES
Wang, L., Tang, T.B., Johannessen, E., Astaras, A.,2002.
An Integrated Sensor Microsystem for Industrial and
Biomedical Applications. IEEE Instrument and
Measurement, [online]. Available from:
http://www.see.ed.ac.uk/~aa/WanTanJohAst02b.pdf
[cited 18 June 2007].
Richards Grayson, A., Shawgo, R., Johnson, A., Flynn, N.,
Li, Y., Cima, M., Langer, R., 2004. A BioMEMS
Review: MEMS Technology for Physiologically
Integrated Devices. Proceedings of the IEEE, [online].
92 (1), pp 6-20.
Kundert, K., Chang, H., Jefferies, D., Lamant, G.,
Malavasi, E., Sendig,F., 2000. Design of Mixed-Signal
Systems-on-a-chip. IEEE Transaction on Computer
Aided Design of Integrated Circuits and Systems. 19
(12), pp 1561-1571.
Matthews, D. J., Gaynor, M. P., 2003. RF System in
Package: Considerations, Technologies and Solutions.
Amkor Technologies. [online]. Available from:
www.amkor.com/products/notes_papers/RF_SiP_Pape
r041403.pdf, [cited 18 June 2007]
Advanced Assembly and Packaging for Biomedical
Devices. Fraunhofer Institut Zuverlassigkeit und
Mikrointegration. [online].Available from:
http://www.pb.izm.fhg.de/izm/040_Publi_News/index.
html, [cited 18 June 2007]
Pai, R., Roussel, T., Crain, M., Jackson, D., Conklin, J.,
Baldwin, R., Keynton, R., Naber, J., Walsh, K., 2001.
Integrated Electrochemical Detection for Lab on a
Chip Analytical Microsystems. Proceedings of the
Fourteenth Biennial University/Government/Industry
Micro electronics Symposium. pp 167-170.
Zhang, J., Manson, A., 2007. Highly Adaptive Transducer
Interface Circuit for Multiparameter Microsystems.
IEEE Transactions on Circuits and Systems. 54 (1), pp
167-177.
Callister, 2003. Materials Science and Engineering and
Introduction 6
th
ed., Wiley & Sons, USA.
Egloff, E. R., The Art of Design and Manufacture of
Polymer Thick Film Circuits. Screen Printing and
Graphic Imaging Association International Technical
Guidebook. [online]. Available from:
www.sgia.org/pdf_server.cfm?pdf=/members/tgbArch
ive/2028.pdf, [cited 19 June 2007].
McKay, W., Gregson, P., McKay, B., Militzer, J., 1999.
Sternal Acceleration Ballistocardiography and Arterial
Pressure Wave Analysis to Determine Stroke Volume.
Clin Invest Med. 2 (1), pp 4-14.
MULTIPARAMETER SINGLE LOCUS INTEGRATED MULTILAYER POLYMER MICROSENSOR SYSTEM
43