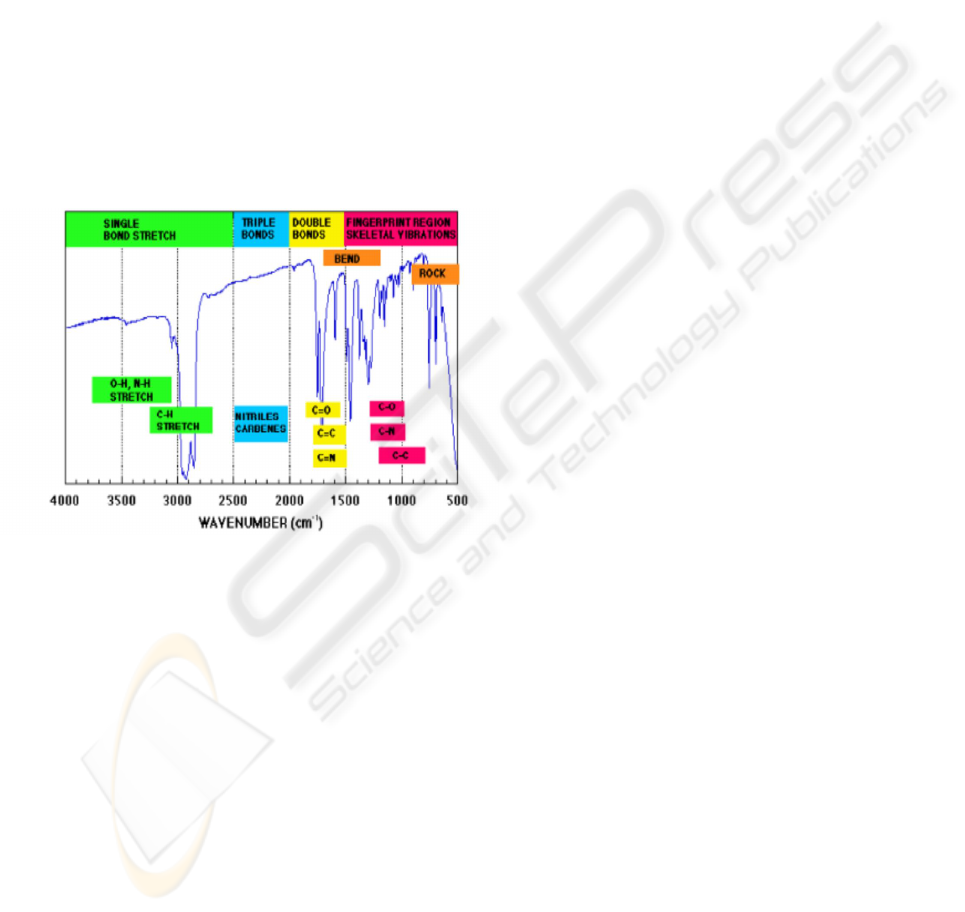
the fiber using a coupling cell: a sensor able to
detect C-H stretch band of ethyl chloride gas has
been realized in this way (975 cm
-1
, Charlton, 2005).
THz lasers, which have higher wavelengths, have
been used for gas phase spectroscopy (Hübers,
2006).
For laser spectroscopy of larger molecules with
broad absorption features narrow linewidth is not
required, but it is more important to be able to tune
the emission over a wide wavelength range. In this
case, the bound-to-continuum structures we
presented provide the required broad spectrum (the
lower state of the transition is a relatively broad
continuum). The fine tuning is achieved by mean of
the utilization of an external cavity configuration:
the emission of the laser is collimated and reflected
off a diffraction grating so to create a resonant
cavity. The first order diffraction from the grating
provides the laser feedback, while the output of the
Figure 9: Absorption regions in the infrared.
external cavity is obtained by the zeroth order. The
tunability is provided by moving and rotating the
grating with piezo actuated positioners. The method
has been proved to be fruitful (Wysocki, 2005) and
it is currently exploited for measuring absorption
spectra of large molecules, like Freon (around 1150
cm
-1
, Phillips, 2007).
Another idea which can be exploited comes from
the realization of surface-emitting quantum cascade
micro cavity lasers: the active region of the laser is
covered with a patterned surface, like a photonic
crystal. The presence of the crystal provides both
feedback for the laser action and a selection of the
polarization of the emitted light (Colombelli, 2003).
This structure could allow also the possibility to
allocate defects on the photonic crystal which can
absorb chemical species: the change in emission due
to the absorption of molecules can be monitored as a
change of the laser emission and the whole would
perform as a compact and sensitive biosensing
device.
6 CONCLUSIONS
QCL present themselves as reliable sources for IR
range: they provide high optical IR power, their
emission can be tailored to span a selected range and
by mean of a superimposed grating it could be made
monomode. The emission is also tuneable with very
fine precision, either intrinsically by changing the
laser temperature or externally by using a coupled
diffraction grating Their performances make them
suitable for a series of application, since most of the
molecular species present distinctive absorption
lines in the medium infrared.
Different methods to realize QCL-based
biosensor have been already tested or are currently
in development, principally in the field of the gas
sensing for medical diagnostics applications or
environmental control: several substances have been
already proved to be detectable through the
utilization of QCL based sensing devices, which
start to provide significant and interesting results.
Such a development is a very interesting field
which looks to be double faced. From one side a
new class of biosensors could profit of the unique
properties of QCL in the IR range: the versatility of
such structures could allow a very wide range of
design and applications. On the other hand the study
of such sensors could provide a further push to the
design and development of even more efficient QCL
structures properly aimed to better match the
requirements of biosensor field.
ACKNOWLEDGEMENTS
The present work has been performed in cooperation
with the SISTER project of the AREA Science Park
of Trieste, Italy.
REFERENCES
Faist J. (1994) ‘Mid-infrared field-tunable intersubband
electroluminescence at room temperature by phonon-
assisted tunneling in coupled-quantum wells’, Applied
Physics Letters, vol. 64 no. 9, February, pp. 1144-
1146.
Hofstetter D. (2001) ‘High temperature operation of
distributed feedback quantum-cascade lasers at 5.3
µm’, Applied Physics Letters, vol. 78 no. 4, January,
pp. 396-398.
QUANTUM CASCADE LASERS FOR BIOSENSING APPLICATIONS
91