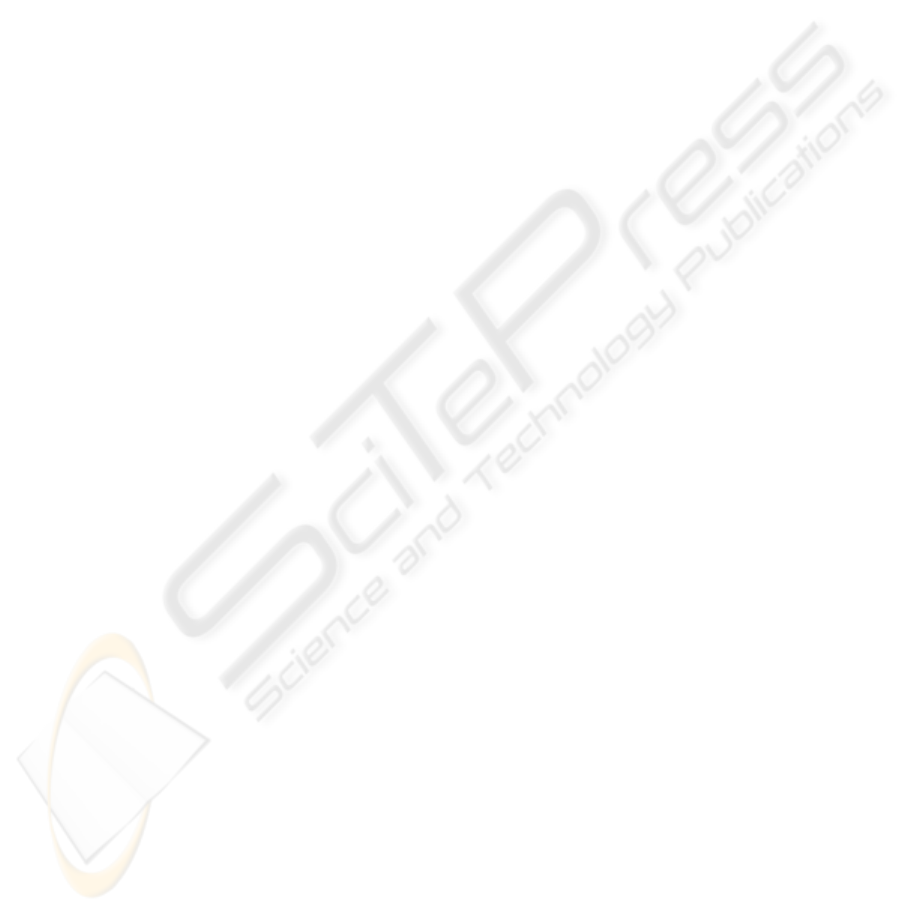
REFERENCES
Allen, R., Foster, M., Yen, Y.-T., 1982. Deep U.V.
Hardening of positive photoresist patterns. J
Electrochem. Soc., 128, 1379.
Caillot-Augusseau, A., Lafage-Reoust, M.-H., Soler, C.,
Pernod, J., Dubois, F., Alexander, C., 1998. Bone
formation and resorption biological markers in
cosmonauts during and after a 180-day space flight
(Euromir 95). Clin. Chem. 44(3), 578-585.
Donahue, H. J., Zhou, Z., Li, Z., McCauley, L. K., 1997.
Age-related decreases in stimulatory G protein-
coupled adenylate cyclase activity in osteoblastic cells.
Am. J. Physiol. 273, E776-E781.
Elderstig, H., Larsson, O., 1997. Polymeric MST-high
precision at low cost. J. Micromech. Microeng., 7, 89-
92.
Fritton, S. P., McLeod, K. J., Rubin, C.T., 2000.
Quantifying the strain history of bone: spatial
uniformity and self-similarity of low-magnitude
strains. J. Biomechanics. 33 (3), 317-325.
Kunz, R. R., 2004. Photoresist outgassing: a potential
Achilles heel for short wave-length optical lithography?
in Proc. of SPIE, 5376, 1-15.
Scuor, N., Gallina, P., Panchawagh, H. V., Mahajan, R.
L., Sbaizero, O., 2006. Design of a novel MEMS
platform for the biaxial stimulation of living cells.
Biomed. Microdevices, 8, 239-246.
Selvaganapathy, P., Leung Ki, Y.-S., Renaud, P.,
Mastrangelo, C. H., 2002. Bubble –free electrokinetic
pumping. J. Microelectromech. Syst., 11(5), 448-453.
Timoshenko, S.P. and Goodier, J.N., 1970. Theory of
Elasticity. 3rd ed. New York: McGraw-Hill Publishing
Company. 409-415.
U.S. Department of Health and Human Services, (2007).
Bone Health and Osteoporosis: A Report of the
Surgeon General. Retrieved July 9, 2007, from
http://www.surgeongeneral.gov/library/bonehealth/fac
tsheet2.html
Yang, S., Saif, T., 2006. MEMS based sensors for the
study of indentation response of single cells. in Tech.
Dig. of MEMS 2006, Istanbul, Turkey, 20-23.
You, J., Yellowley, C. E., Donahue, H. J., Jacobs, C. R.,
1999. Physiological levels of substrate deformation are
less stimulatory to bone cells compared to fluid flow,
In American Society of Mechanical Engineers,
Bioengineering Division (Publication) BED, 43, 161-
162.
You, L., Cowin, S. C., Schaffler, M. B., Weinbaum, S.,
2001. A model for strain amplification in the actin
cytoskeleton of osteocytes due to fluid drag on
pericellular matrix. J. Biomechanics. 34 (11), 1375-
1386.
Zhang, W.-Y., 2007. Design, Modeling, Fabrication and
Characterization of a MEMS Device for Measuring
the Mechanical Compliance of a Biological Cell. Ph.D.
Dissertation, Lehigh University, 2007.
BIODEVICES 2008 - International Conference on Biomedical Electronics and Devices
150