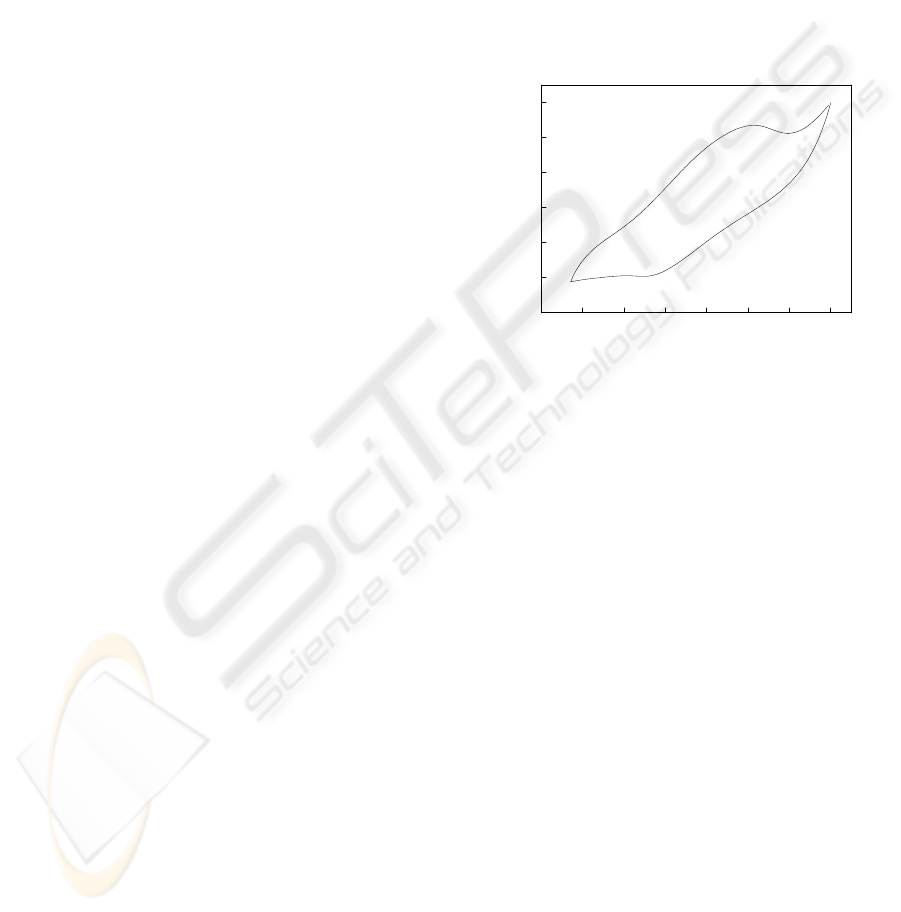
and deaerated. Voltammetric measurements were
performed with a micro-Autolab Type III controlled
by an appropriated software.
2.2 Reagents and Solutions
All solutions were prepared using a Millipore Milli-
Q water. All chemicals were analytical reagent grade
and were used without further purification. The
supporting electrolyte used for most of the
experiments was a 0.1 mol l
-1
Tris buffer solution
(pH = 8.30). A 0.01 mol l
-1
potassium ions solution
was prepared daily by dissolving potassium chloride
(Merck) in 100 ml of such Tris buffer solution.
Graphite powder (1–2 μm particle size from
Aldrich) and mineral oil (Aldrich) of high purity
were used for the preparation of the sensor.
2.3 Preparation of Hollandite-Type
MnO
2
Hollandite-type manganese(IV) oxide was prepared
using a reflux method according to literature (Ching
et al. 1997). For conversion to hollandite-type
manganese oxide, a KMn
2
O
4
sample was treated in
an aqueous diluted sulfuric acid solution kept under
constant stirring during 120 min. When the pH of
this mixture was stabilized at a particular value, the
solution was decanted and the remaining solid
material washed by decantation with deionized
water, filtered and dried in air at 90
ºC.
2.4 Sensor Construction
Sensors with hollandite-type MnO
2
were prepared
by carefully mixing the dispersed graphite powder
with manganese oxide
at varying ratio. Exactly 1 g
of this mixture was subsequently added to 0.200 g of
mineral oil (20% m/m) and mixed in a 50 ml beaker
containing 20 ml of hexane. The final paste was
obtained with the solvent evaporation. The carbon-
paste electrode was finally obtained packing the
paste into a plastic tube (1 ml insulin plastic syringe)
and arranged with a copper wire serving as an
external electric contact.
3 RESULTS AND DISCUSSION
3.1 Electrochemical Behavior
First, the voltammetric behavior of the CPEM with
hollandite-type manganese oxide in Tris buffer
solution (pH 8.3) containing 5.0 x 10
-4
mol L
-1
potassium ions was investigated. The cyclic
voltammograms obtained with the sensor (see Fig.
1) presented one anodic peak (peak I = 0.63 V vs.
SCE) and another cathodic peak (peak II = 0.08 vs.
SCE). This electrochemical activity is due to the
extraction topotactic process of the potassium ions
from the hollandite structure, which occurs in two
steps to the electrochemical insertion/extraction
processes of the potassium ions (Feng et al., 1995):
4
4
2
3
OMnMnK
+
−
+
xxx
(s)
→ 2.MnO
2(s)
+ x K
+
(aq)
+ x e
-
(1)
2.MnO
2(s)
+ x K
+
(aq)
+ x e
-
→
4
4
2
3
OMnMnK
+
−
+
xxx
(s)
(2)
-0.2 0.0 0.2 0.4 0.6 0.8 1.0
-200
-100
0
100
200
300
peak II
peak I
I / μA
E (V) vs. ECS
Figure 1: Cyclic voltammogram of the sensor in 5.0 x 10
-4
mol L
−1
of potassium ions, at a scan rate of 50 mV s
−1
between − 0.15 and 1.00 V versus SCE.
The enhanced response to potassium ions occurs
because the cathodic polarization of the sensor with
hollanditel-type manganese oxide is quite enough to
reduction the manganese in the solid. Consequently,
the potassium ions from the adjacent solution are
able to diffuse though the hollandite structure to
maintain the electroneutrality principle.
In the absence of potassium ions, no voltammetric
response was observed for the sensor, confirming
that the response of the sensor is a function of the
insertion reaction of potassium ions in the hollandite
structure as mentioned previously (see Eq. 3):
2.MnO
2(s)
+ x K
+
(aq)
+ (x/2) H
2
O
(l)
→
4
4
2
3
OMnMnK
+
−
+
xxx
(s)
+ x H
+
(aq)
+ (x/4) O
2(g)
(0 < x < 1)
(3)
where is vacant site (tunnel) of the manganese
oxide.
The apparent electrochemical rate constant k
e and
the electron-transfer coefficient α
anodic
were calculated
for the sensor according to the method described by
Larivon (Larivon, 1979). It has been shown by
Laviron that for a surface redox couple, α
anodic
and ke
can be determined from the variation of Epa with
scan rate. Figure 2 presents the plot of E
pa (V)
DEVELOPMENT OF AN AMPEROMETRIC SENSOR FOR POTASSIUM IONS
199