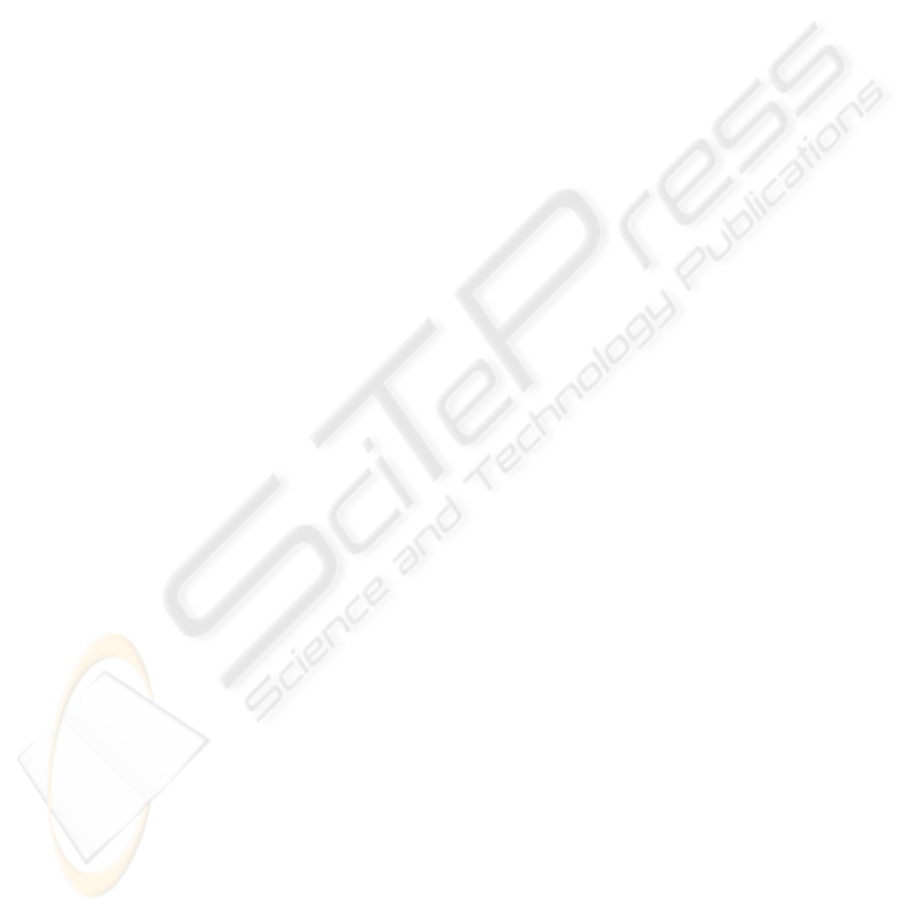
resulting bandwidth may be outside the structure
bandwidth due to the instantaneous required
frequency shifts. In this way, a continuous
modulating method must be used instead.
To check the ability to operate as a radiating
element, some preliminary tests were also
conducted, where it was used a scaled model of the
proposed structure. A commercially available
magnetic sensor was connected to a signal
acquisition board that was connected to a personal
computer. A current was injected into a scaled
structure of Fig. 4, and the signal was recorded with
the magnetic sensor. When the transmitting structure
was oscillating at 100 KHz, it was possible to easily
detect that signal with the magnetic sensor.
5 CONCLUSIONS
This paper described the design, and modelling of
chip-size MEMS antennas for short-range wireless
microsystems. These antennas allow the fabrication
of an implantable microsystem with integrated
wireless communications. The antenna integration is
based on wafer-level packaging techniques, which
enables the integration of new materials with the
standard silicon processing steps, as well the
fabrication of complex three-dimensional structures,
in an economically acceptable way.
MEMS were explored as a new solution to
obtain structures that can sense electromagnetic
fields. Thus, instead of having the need to design
very advanced antenna structures to achieve antenna
size reduction, the standard MEMS devices, e.g.
cantilevers, will be used to save system space and
improve system integration. A novel electrically
very small antenna using MEMS structures, and a
model to describe the operation of that structure; is
presented.
The present solution envisions power saving,
smaller volume, lower cost, and increased system
lifetime, which are very important features in
biomedical microsystems for diagnosis and therapy.
ACKNOWLEDGEMENTS
We would like to acknowledge Medical Doctor
Paulo Vale for his support in this research work.
REFERENCES
Carmo, J. P., Mendes, P..M., Couto, C., Correia, J.H.,
2006, “5.7 GHz on-chip antenna/RF CMOS
transceiver for wireless sensors network,” Journal
Sensors and Actuators A, Elsevier Science, Vol. 132,
pp. 47-51,
Gaunt, R. A, and Prochazka, A., 2005, “Control of urinary
bladder function with devices: successes and failures,”
Progress in Brain Research, Elsevier, Vol. 152, pp.
163-194.
Keplinger, F., Kvasnica, S., Jachimowicz, A., Kohl, F.,
Steurer, J., and Hauser, H., 2004, “Lorentz force based
magnetic field sensor with optical readout,” Sens.
Actuators A, 110, (1–3):112–118.
Kitchen, R, 1993, RF Radiation Safety Handbook,
Butterworth-Heinemann.
Lange, D., Brand, O., and Baltes, H., 2002, CMOS
Cantilever Sensor Systems: Atomic Force Microscopy
and Gas Sensing Applications., Springer.
Mendes, P. .M., and Coreeia, J. H., 2007, "MEMS Micro-
Antennas for Wireless Biomedical Systems," in
"Wireless Communications Research Trends", ISBN:
1-60021-674-9, 2007, edited by Tong S. Lee, Nova
Publishers.
Piella, J. P., 2001, Energy management, wireless and
system solutions solutions for highly intregated
implantable devices. PhD Thesis, Universitat
Autónoma de Barcelona, Barcelona.
Rocha, L. A., Cretu, E., Wolffenbuttel, R. F. 2004,
“Analysis and Analytical Modeling of Static Pull-In
With Application to MEMS-Based Voltage Reference
and Process Monitoring,” Journal Of
Microelectromechanical Systems, Vol. 13, No. 2, pp.
342-354.
MEMS ANTENNA FOR WIRELESS BIOMEDICAL MICROSYSTEMS - Extremely Small Antenna for RF Receivers in
Implantable Devices
75