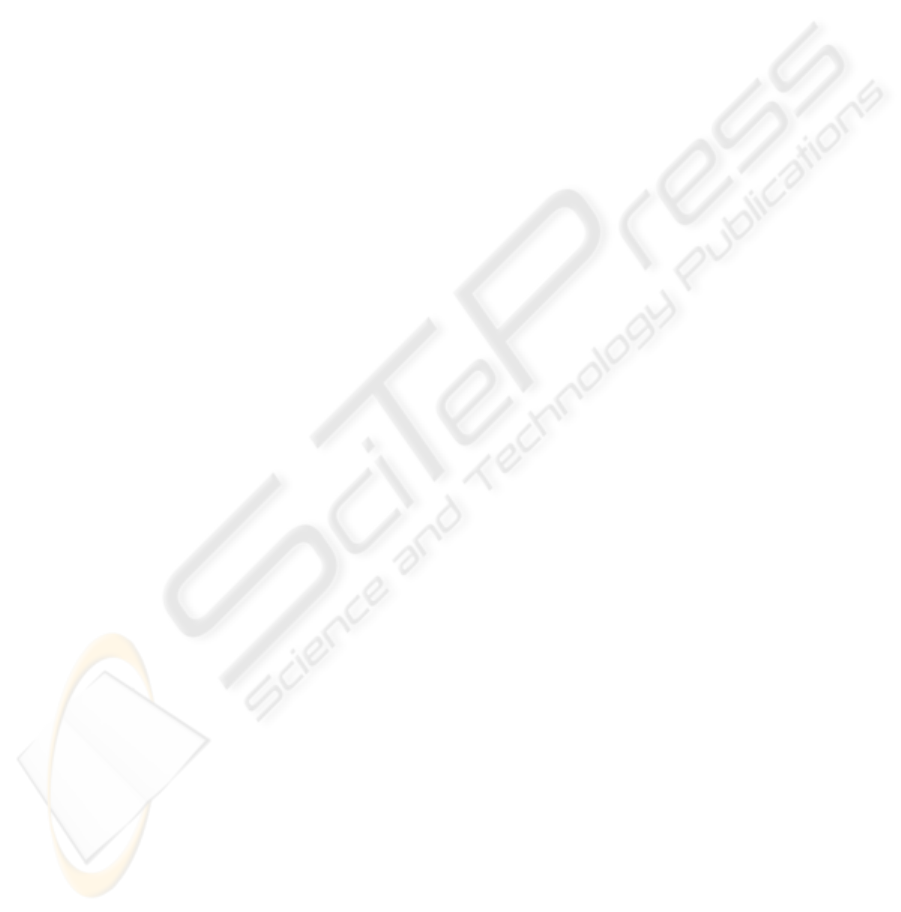
printing operating parameters are both key to the
success of ink jet printing functional materials.
Established operating parameters can now be
translatable to production line systems with built in
versatility, uniformity and scalability for biodevice
production. Future directions will be to incorporate
ink jet printing with circuit printing and biological
fluid deposition for single deposition biodevice
processing methods.
REFERENCES
Antoniadis, H. Low-cost solar cells exploiting solar ink. in
IEEE San Francisco Bay Area Nanotechnology
Council Monthly meeting, 2007. Santa Clara, CA,
USA.
Bae, K.D., et al., Development of the new thermal head on
SOI wafer. Microelect. Eng, 2005. 78-79: p. 158-163.
Baiesi, M., et al., Zipping and collapse of diblock
copolymers. Physical Review E, 2001. 63: p. 41801-
41811.
Brünahl, J. and A.M. Grishij, Piezoelectric shear mode
drop-on-demand ink jet actuator. Sens. Act. A, 2002,
101:371-382.
Calvert, P., Ink jet printing for materials and devices.
Chem. Mater. , 2001. 13: p. 3299-3305.
Diehl, F., et al., Manufacturing DNA microarrays from
unpurified PCR products. Nucleic Acids Res., 2002.
30: p. 79-84.
Grishij, Piezoelectric shear mode drop-on-demand ink jet
actuator. Sens. Act. A, 2002. 101: p. 371-382.
Haber, C., M. Boillat, and B. van der Schoot, Precise
nanoliter fluid handling system with integrated high-
speed flow sensor. Assay Drug Dev Technol, 2005.
3(2): p. 203-12.
Hwang, J.S., Solder materials and process for electronic
assembly fabrication, in Electronic Assembly
Fabrication, chips, circuit boards, packages and
components, C.A. Harper, Editor. 2002, McGraw-Hill:
New York, NY. p. 305-362.
Lemmo, A.V., D.J. Rose, and T.C. Tisone, Ink jet
dispensing technology: applications in drug discovery.
Curr. Opin. Biotechnol., 1998. 9: p. 615-617.
Lewis, J.A., et al., Direct ink writing of three-dimensional
ceramic structures. J. Am. Ceram. So, 2006. 89: p.
3599–3609.
Manning, H., Application of ink jet technology for
miroarrays and other bio printing, in Bioprinting.
2007: London, England.
Mei, J., M.R. Lovell, and M.H. Mickle, Formulation and
processing of novel conductive solution inks in
continuous ink jet printing of 3-D electric circuits.
IEEE Trans. Electron. Packag. Manuf., 2005. 28: p.
265-273.
Menzel, C. MEMS solutions for precision micro-fluidic
dispensing application. in NIP20: International
Conference on Digital Printing Technologies. 2005.
Salt Lake City Utah.
Myatt, C., N. Traggis, and K.L. Dessau, Optical
fabrication: optical contacting grows more robust.
Laser Focus World, 2006. 42: p. 95-98.
Padinger, F., Biosensors and printed electronics for life
sciences, in Bioprinting 2007: London, England.
Peck, W., in-situe microarray manufacturing using ink jet
technology at Agilent Technologies, in Bioprinting.
2007: London, England.
Rodriguez-Calvo, M.S., et al., Isolectric focusing of
human hair keratins: correlation with sodium dodecyl
sulfate-polyacrylamide gel electrophoresis (SDS-
PAGE): patterns and effect of cosmetic treatments. J.
Forensic Sci., 1992. 37: p. 425-431.
Salas, A., et al., Fluorescent SSCP of overlapping
fragments (FSSCP-OF): a highly sensitive method for
the screening of mitochondrial DNA variation.
Forensic Sci. Inter., 2001. 124: p. 97-103.
Setti, L., et al., An amperometric glucose biosensor
prototype fabricated by thermal ink jet printing.
Biosensors and Bioelectronics, 2005. 20: p. 2019-
2026.
Sawhney, A., et al., Piezoresistive sensors on textiles by
ink jet printing and electroless plating. Mater. Res.
Symp. Proc., 2006. 920: p. 4-13.
Shaw, J.M. and P.F. Seidler, Organic electronics:
Introduction. IBM Journal of Research and
Development, 2001. 45(1): p. 3-10.
Sirringhaus, H., et al., High-resolution ink jet printing of
all-polymer transistor circuits. Science, 2000.
290(5499): p. 2123-6.
Song, J.H. and H.M. Nur, Defects and prevention in
ceramic components fabricated by ink jet printing.
Mater. Proc. Technol. , 2004. 155: p. 1286-1292.
Sumerel, J., et al., Cyclin E and its associated cdk activity
do not cycle during early embryogenesis of the sea
urchin. Dev Biol., 2001. 234(2): p. 425-40.
Sumerel, J., et al., Piezoelectric ink jet processing of
materials for medical and biological applications.
Biotechnol J, 2006. 1(9): p. 976-87.
Teng, K.F. and R.W. Vest, Metallization of solar cells
with ink jet printing and silver metallo-organic inks.
IEEE Trans. Compon. Hyb. Manuf. Tech., 1988. 11:
p. 291-297.
Volkman, S.K., et al., Ink-jetted silver/copper conductors
for printed RFID applications. Mat. Res. Soc. Symps.
Proc., 2004. 814: p. 1-6.
Vuorio, A.F., et al., Amplification of the hypervariable
region close to the apolipoprotein B gene: application
to forensic problems. Biochem. Biophys. Res. Comm.
, 1990. 170: p. 616-620.
Wilson, M.R., et al., Validation of mitochondrial DNA
sequencing for forensic casework analysis. Int. J.
Legal. Med. , 1995. 108: p. 68-74.
Xu, T., et al., Ink jet printing of viable mammalian cells.
Biomaterials, 2005. 26(1): p. 93-9.
JUST PUSH PRINT - Biodevice Printing Using Bioinks, Electroinks and Quantum Dot Inks
303