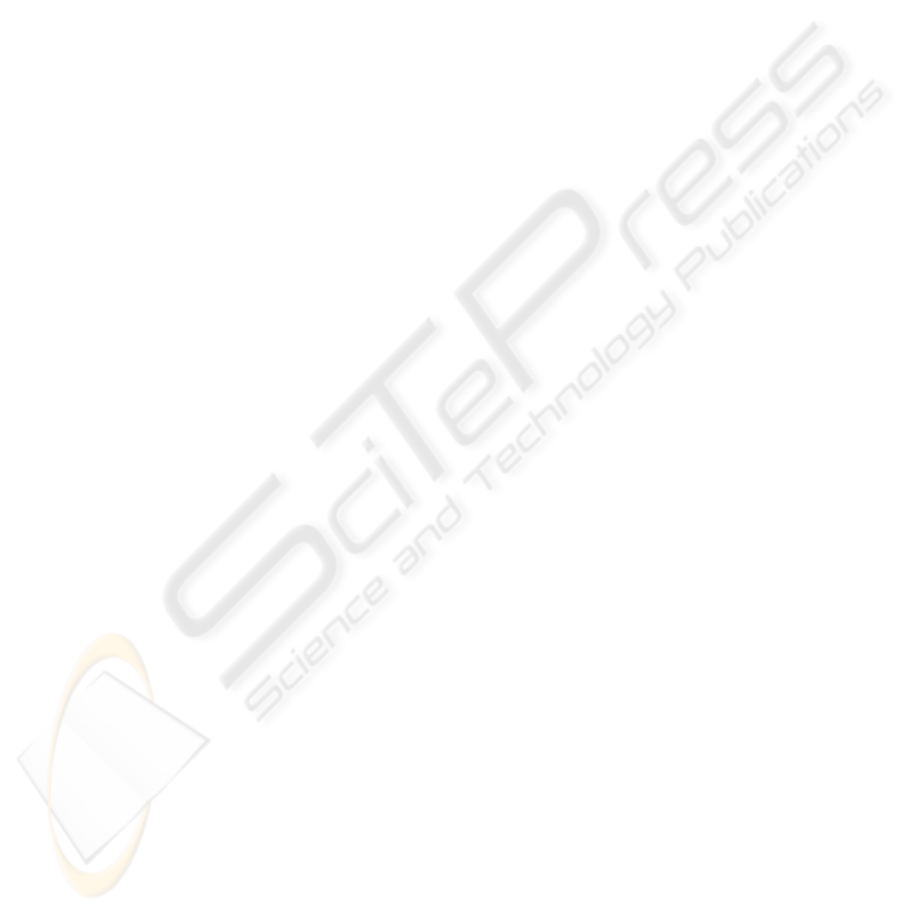
REFERENCES
Bousse, L. (1982). The Chemical Sensitivity of Elec-
trolyte/Insulator/Silicon Structures. PhD thesis.
Bousse, L., Mostarshed, S., Van Der Shoot, B., De Rooij,
N. F., Gimmel, P., and Gopel, W. (1991). Zeta po-
tential measurements of Ta
2
O
5
and SiO
2
thin films.
Journal of Colloid and Interface Science, 147(1):22–
32.
Cui, Y., Wei, Q., Park, H., and Lieber, C. M. (2001).
Nanowire nanosensors for highly sensitive and selec-
tive detection of biological and chemical species. Sci-
ence, 293(5533):1289–1292.
Deen, M. J. (2007). Highly sensitive, low-cost integrated
biosensors. In SBCCI 2007: 20th Symposium on Inte-
grated Circuits and System Design, page 1.
Deen, M. J., Shinwari, M. W., Ranu´arez, J. C., and
Landheer, D. (2006). Noise considerations in field-
effect biosensors. Journal of Applied Physics,
100(7):074703–1 –074703–8.
Fritz, J., Cooper, E. B., Gaudet, S., Soger, P. K., and Man-
alis, S. R. (2002). Electronic detection of DNA by
its intrinsic molecular charge. In PNAS, volume 99,
pages 1412–1416.
Gao, Z., Agarwal, A., Trigg, A., Singh, N., Fang, C., Tung,
C., Fan, Y., Buddharaju, K., and Kong, J. (2007). Sil-
icon nanowire arrays for label-free detection of DNA.
Analytical Chemistry, 79(9):3291–3297.
Girard, A., Bendria, F., Sagazan, O. D., Harnois, M., Bihan,
F. L., Sala¨un, A., Mohammed-Brahim, T., Brissot, P.,
and Lor´eal, O. (2006). Transferrin electronic detec-
tor for iron disease diagnostics. IEEE Sensors, pages
474–477.
Gupta, S., Elias, M., Wen, X., Shapiro, J., and Brillson,
L. (2008). Detection of clinical relevant levels of
protein analyte under physiologic buffer using planar
field effect transistors. Biosensors and Bioelectronics,
24:505–511.
Hahm, J. and Lieber, C. M. (2004). Direct ultrasensitive
electrical detection of DNA and DNA sequence vari-
ations using nanowire nanosensors. Nano Letters,
4(1):51–54.
Heitzinger, C., Kennell, R., Klimeck, G., Mauser, N.,
McLennan, M., and Ringhofer, C. (2008a). Modeling
and simulation of field-effect biosensors (BioFETs)
and their deployment on the nanoHUB. J. Phys.:
Conf. Ser., 107:012004/1–12.
Heitzinger, C. and Klimeck, G. (2007). Computational as-
pects of the three-dimensional feature-scale simula-
tion of silicon-nanowire field-effect sensors for DNA
detection. Journal of Computational Electronics,
6:387–390.
Heitzinger, C., Mauser, N., and Ringhofer, C. (2008b).
Multi-scale modeling of planar and nanowire field-
effect biosensors. submitted.
http://www.pdb.org.
Im, H., Huang, X. ., Gu, B., and Choi, Y. . (2007).
A dielectric-modulated field-effect transistor for
biosensing. Nature Nanotechnology, 2(7):430–434.
Kim, D., Park, J., Shin, J., Kim, P., Lim, G., and Shoji, S.
(2006). An extended gate FET-based biosensor inte-
grated with a Si microfluidic channel for detection of
protein complexes. Sensors and Actuators, B: Chemi-
cal, 117:488–494.
Kusnezow, W., Jacob, A., Walijew, A., Diehl, F., and Ho-
heisel,J.D. (2003). Antibody microarrays: An evalua-
tion of production parameters. Proteomics, 3(3):254–
264.
Landheer, D., Aers, G., McKinnon, W., Deen, M., and
Ranu´arez, J. (2005). Model for the field effect from
layers of biological macromolecules on the gates of
meta-oxide-semiconductor transistors. journal of ap-
plied physics, 98(4):044701–1 –044701–15.
Oh, S. W., Moon, J. D., Lim, H. J., Park, S. Y., Kim, T.,
Park, J., Han, M. H., Snyder, M., and Choi, E. Y.
(2005). Calixarene derivative as a tool for highly
sensitive detection and oriented immobilization of
proteins in a microarray format through noncovalent
molecular interaction. FASEB Journal, 19(10):1335–
1337.
Park, K., Lee, S., Sohn, Y., and S.Y, C. (2008). BioFET
sensor for detection of albumin in urine. Electronic
Letters, 44(3).
Peluso, P., Wilson, D. S., Do, D., Tran, H., Venkatasub-
baiah, M., Quincy, D., Heidecker, B., Poindexter, K.,
Tolani, N., Phelan, M., Witte, K., Jung, L. S., Wagner,
P., and Nock, S. (2003). Optimizing antibody immo-
bilization strategies for the construction of protein mi-
croarrays. Analytical Biochemistry, 312(2):113–124.
Pirrung, M. C. (2002). How to make a DNA chip. Angew.
Chem. Int. Ed., 41:1276–1289.
Poghossian, A., Cherstvy, A., Ingebrandt, S., Offenh¨ausser,
A., and Sch¨oning, M. J. (2005). Possibilities and lim-
itations of label-free detection of DNA hybridization
with field-effect-based devices. Sensors and Actua-
tors, B: Chemical, 111-112(SUPPL.):470–480.
Ringhofer, C. and Heitzinger, C. (2008). Multi-scale mod-
eling and simulation of field-effect biosensors. ECS
Transactions, 14(1):11–19.
Selberherr, S. (1984). Analysis and Simulation of Semi-
conductor Devices, volume Springer of ISBN: 3-211-
81800-6.
Shinwari, M. W., Deen, M. J., and Landheer, D. (2006).
Study of the elecrolyte-insulator-semiconductor field-
effect transistor (EISFET) with applications in biosen-
sor design. Microelectronics Reliability.
Stern, E., Klemic, J., Routenberg, D., Wyrembak, P.,
Turner-Evans, D., Hamilton, A., LaVan, D., Fahmy,
T., and Reed, M. (2007). Lable-free immunodetection
with CMOS-compatible semiconducting nanowires.
Nature Letters, 445(1):519–522.
Tang, T.-W. and Ieong, M.-K. (1995). Discretization of
flux densities in device simulations using optimum ar-
tificial diffusivity. IEEE Transactions on Computer-
Aided Design of Integrated Circuits and Systems,
14(11):1309–1315.
Turkova, J. (1999). Oriented immobilization of biologically
active proteins as a tool for revealing protein interac-
tions and function. Journal of Chromatography B:
STUDY OF THE PROPERTIES OF BIOTIN-STREPTAVIDIN SENSITIVE BIOFETS
29