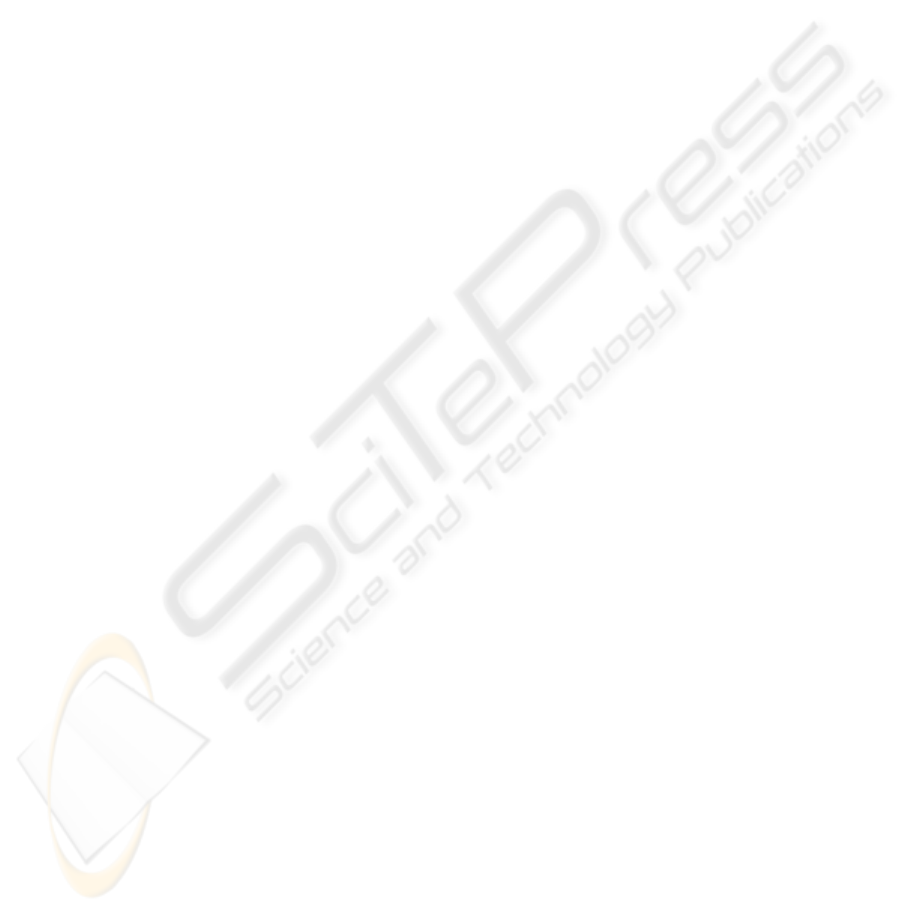
Figure 12 shows the results for the same stimulus
train but with the coil placed over Brodmann area 9.
Two features can be noted: (i) the F3 channel is not
saturated since the coil was not immediately over the
F3 electrode position and the pattern of EPs is
different for that shown in Figure 11.
4 CONCLUSIONS
Our research has shown that standard commercial
Au and Ag or Ag-AgCl EEG electrodes cannot be
used for general rTMS applications, due to excessive
skin heating. However, these electrodes can be used
if fully or “C” notched. Further the choice among
these electrodes does not seem to affect the
amplitude or duration of the stimulus artefact.
Although our heating results are in general
agreement with the conclusions of previous
researchers, the lack of dependency of the stimulus
artefact amplitude on electrode material does not
(Virtanen et al, 1999). Their results show that the
amplitude depends mostly on electrode size and that
Ag-AgCl electrodes had very low artefacts
compared to Ag, although this could be a result of
the very small Ag-AgCl pellet size. Further, their
fully notched Ag standard electrodes had much
lower artefact than the intact ones. The principle
contributors to the stimulus artefact are not well
understood, and electrode, wire and input amplifier
capacitances all play a part. Even in a multichannel
recording situation, where the magnetic field
orientation and amplitude is very different for each
electrode, the residual stimulus artefacts can be very
similar as shown in Figure 8. Further research will
be conducted to investigate the determining factors
for magnetically induced artefacts and how common
these are for all stimulating and recording
conditions.
The new EEG system works very well, and
depending on the stimulus strength, the amplifier
can be reconnected to the recording electrodes with
delays from 1 to 4 msec, allowing us to record EPs
as well as ERPs. The initial voltage offset
introduced by sample and hold circuitry, shown as
the square pulse in Figures 8 and 9, can be ignored
and does not affect the signal when the block is
terminated. Tests with the stimulating coil at some
distance from the electrodes resulting in very low
short duration artefacts have shown that the
amplitude returns to baseline within μsec. The test
results shown in this paper were for worst case
scenario experiments with large stimulus amplitudes
and the coil either directly over the recording
electrodes or in close proximity. However, the
system must be made more immune to
environmental noise by better shielding and cabling.
Future work will include postprocessing to
estimate and remove the exponentially decaying
residual artefact and periodic environmental noise.
Although synchronous averaging can remove
asynchronous environmental noise if enough stimuli
are given, the technique is inefficient. The number
of stimuli presented to the brain should be
determined by clinical efficacy rather than noise
reduction.
The multi-channel results show that even when
stimulus artefact is removed new signal processing
techniques will have to be developed to model and
remove the muscle M-waves.
ACKNOWLEDGEMENTS
Support for this research from the Natural Sciences
and Engineerung Research Council of Canada is
gratefully acknowledged.
REFERENCES
Archambeault, M., de Bruin, H. 2007, Development of an
electroencephalography data acquisition system for
clinical research into transcranial magnetic stimultion
evoked potentials, Proc. IEEE Cdn. Conf. ECE,
Vancouver, pp 202 – 205.
Fitzgerald P.B., Brown, T.L., Marston, N.A., Daskalakis,
Z.J., De Castella, A., Kulkarni, J. 2003, Transcranial
magnetic stimulation in the treatment of depression: a
double-blind, placebo-controlled trial. Arch Gen
Psychiatry 60(10): 1002-1008.
Fuggetta, G., Fiaschi, A., Manganotti, P. 2005, A direct
demonstration of cortical oscillatory activities induced
by varying single-pulse transcranial magnetic
stimulation intensity over the left primary motor area:
a combined EEG and TMS study. Neuroimage 27(4):
896-908
Hoffman, R.E., Gueorguieva, R., Hawkins, K.A.,
Varanko, M., Boutros, N.N., Wu, Y.T., Carroll, K.,
Krystal, J.H. 2005, Temporoparietal transcranial
magnetic stimulation for auditory hallucinations:
safety, efficacy and moderators in a fifty patient
sample. Biol Psychiatry 58(2): 97-104.
Ives, J.R., Rotenburg, A., Poma, R., Thut, G., Pascual-
Leone, A. 2006, Electroencephalographic recording
during transcranial magnetic stimulation in humans
and animals. Clin Neurophysiol 117:1870-1875.
RECORDING EEG DURING REPETITIVE TRANS-CRANIAL MAGNETIC STIMULATION
271