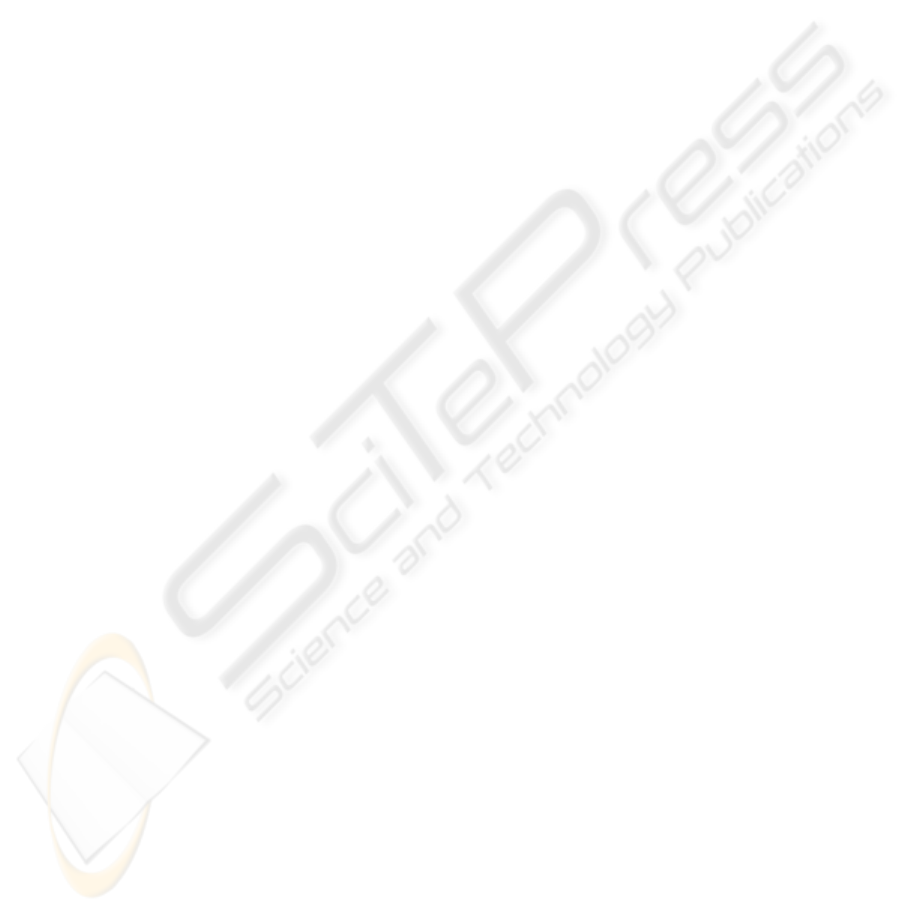
5 CONCLUSIONS
This study shows that the fluctuations of cutaneous
blood flow (cutaneous LDF signals) recorded in
healthy human subjects are different in
hand (glabrous skin) and forearm (non glabrous
skin). These differences are observed in both the
temporal and the spectral domains. Thus, the mean
amplitude of LDF signals recorded in the hand is
generally higher than in the forearm, and the
fluctuations observed in the hand are much higher
than the ones in the forearm. Furthermore, our work
shows that the power spectrum of LDF signals
recorded in hand and forearm of healthy subjects
may be different. They both may possess
characteristics of fractal processes, but these
characteristics are different for the two analyzed
anatomical sites.
In this paper, a monofractal study has been
performed through the power spectral density
analysis. A multifractal analysis could also be
carried out. Multifractal time series are
heterogeneous, self-similar only in local ranges of
the structure and their fractal measure does vary in
time; hence, they can be characterized by a set of
local fractal measures. Some papers have recently
been published on this field of interest for LDF
data (see for example Humeau et al., 2009; Humeau
et al., 2008).
ACKNOWLEDGEMENTS
The authors thank the “Instituto de Investigação
Interdisciplinar (III)” of the University of Coimbra,
“Acções Universitárias Integradas Luso–Francesas”
(PAUILF) programme and “Fundação para a
Ciência e a Tecnologia (FCT), Lisbon”, for
supporting this work.
REFERENCES
Berardesca E., Lévêque J. L. and Masson P. (2002).
EEMCO Guidance for the Measurement of Skin
Microcirculation. Skin Pharmacology and Applied
Skin Physiology, 15, 442-456.
Fredriksson I., Fors C. and Johansson J. (2007). Laser
Doppler Flowmetry - a Theoretical Framework.
Department of Biomedical Engineering, Linköping
University.
Ivanov P. C., Amaral L. A. N., Goldberger A. L., Havlin
S., Rosenblum M. G., Stanley H. E. and Struzik Z. R.
(2001). From 1/f noise to multifractal cascades in
heartbeat dynamics. Chaos, 11(3), 641-652.
Humeau A., Buard B., Chapeau-Blondeau F., Rousseau
D., Mahe G. and Abraham P. (2009). Multifractal
analysis of central (electrocardiography) and
peripheral (laser Doppler flowmetry) cardiovascular
time series from healthy human subjects.
Physiological Measurement, 30, 617-629.
Humeau A., Chapeau-Blondeau F., Rousseau D.,
Rousseau P., Trzepizur W. and Abraham P. (2008).
Multifractality, sample entropy, and wavelet analyses
for age-related changes in the peripheral
cardiovascular system: Preliminary results. Medical
Physics, 35, 717-723.
Lossius K., Eriksen M. and Walloe L. (1992). Fluctuations
in blood flow to acral skin in humans: connection with
heart rate and blood pressure variability. Journal of
Physiology, 460:641-655.
Morales F. (2005). Improving the clinical applicability of
laser Doppler perfusion monitoring. PhD thesis,
Groningen University.
Nilsson G. E., Salerud E. G., Strömberg T. and Wardell K.
(2003). Laser Doppler perfusion monitoring and
imaging, In: Vo-Dinh T (eds) Biomedical photonics
handbook. CRC Press, Boca Raton.
Popivanov D., Mineva A., Dushanova J., 1999, ‘Dynamic
characteristics of laser-Doppler flux data’, Technology
and Health Care, 7:205-218.
Roustit M., Simmons G. H., Carpentier P. and Cracowski
J. L. (2008). Abnormal digital neurovascular response
to local heating in systemic sclerosis. Rheumatology,
47, 860-864.
Roustit M., Blaise S. and Cracowski J. L. (2009). Sodium
iontophoresis on the finger pad does not consistently
increase skin blood flow in healthy controls and
patients with systemic sclerosis. Microvascular
Research, 77(3), 260-264.
Saumet J. L., Degoute C. S., Saumet M. and Abraham P.
(1992). The effect of nerve blockage on forearm and
finger skin blood flow during body heating and
cooling. International Journal of microcirculation,
11(3), 231-40.
Stefanovska A., Bracic M. and Kvernmo H.D. (1999).
Wavelet analysis of oscillations in the peripheral blood
circulation measured by laser Doppler technique.
IEEE Transactions on Biomedical Engineering, 46,
1230–1239.
Thoresen M. and Walloe T. (1980). Skin blood flow in
humans as a function of environmental temperature
measured by ultrasound. Acta Physiologica
Scandinavica, 109, 333-341.
Tucker A. T., Pearson R. M., Cooke E. D. and Benjamin
N. (1998). Effect of nitric-oxide-generating system
on microcirculatory blood flow in skin of patients with
severe Raynaud’s syndrome: a randomized trial. The
Lancet
, 354, 1670-1675.
Wilson T. E., Zhang R., Levine B. D. and Crandall C. G.
(2005). Dynamic autoregulation of the cutaneous
circulation: differential control in glabrous vs. non-
glabrous skin. American Journal of Physiology, Heart
and Circulatory Physiology, 289, H385-H391.
BIOSIGNALS 2010 - International Conference on Bio-inspired Systems and Signal Processing
138