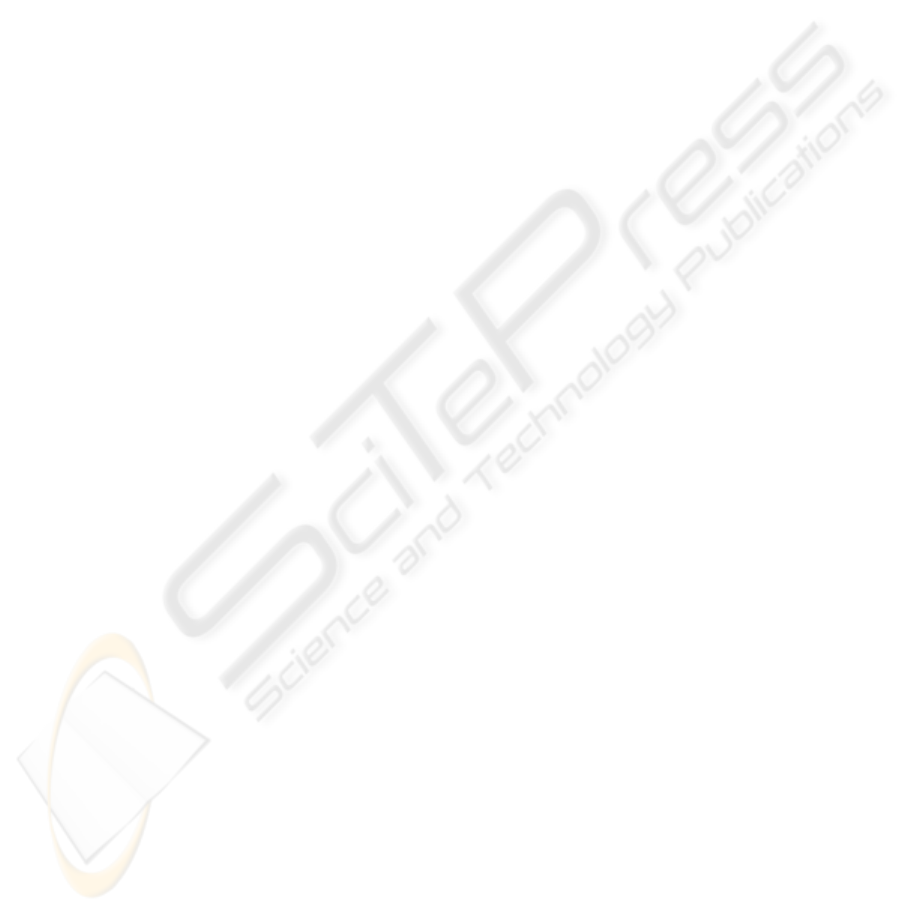
5 CONCLUSIONS
In this study, we have successfully demonstrated the
utility of a tapered electrode structure to
dielectrophoretically dispense variable volume
nanoliter to sub-nanoliter sample droplets (2.4 nL to
0.25 nL) on top of hydrophobic surfaces, with
precision. This tapered droplet scheme was
furthermore interfaced with a fishbone droplet
conveyance scheme to demonstrate its utility in
performing a quantitative, multiplexed assay.
The fluidic sample handling capabilities of the SMF
devices reported in this article may be potentially
leveraged for several purposes including drug
discovery, genomics and pathogen detection. This
SMF scheme can also be multiplexed to an m
n
matrix to achieve HTS capabilities as an alternative
to the existing close channel technology (Thorsen et
al. 2002). The oil bath submerged experimental
setup can be replaced by using the sub-nanoliter
emulsion dispensing scheme, reported by Prakash
and Kaler (2009).
ACKNOWLEDGEMENTS
The authors gratefully acknowledge the financial
support provided by National Science and
Engineering Research Council of Canada (NSERC),
CMC Microsystems and Micralyne Inc. (Canada) in
support of the research work detailed in this article.
Authors furthermore acknowledge the assistance
provided by the Nanofab staff at U of Alberta in
fabricating the SMF devices.
REFERENCES
Ahmed R. and Jones T.B., 2006. Dispensing picoliter
droplets on substrates using dielectrophoresis. J.
Electrostat 64:543–549.
Chugh D. and Kaler K.V.I.S.,2009. Microfluid Nanofluid,
DOI 10.1007/s10404-009-0469-7.
Chugh D. and Kaler K.V.I.S., 2008. Integrated liquid and
droplet DEP for lab-on-a-chip applications.
Proceedings of 12th international conference on
miniaturized systems for chemistry and life sciences,
San Diego, pp 733–735.
Gunji M., Nakanishi H. and Washizu M., 2004. Droplet
actuation based on single-phase electrostatic
excitation. Proceedings of Micro Total Analysis
Systems, Malmo, pp 168–170.
Hong J.W. and Quake S.R., 2003. Integrated nanoliter
systems. Nat. Biotechnology, 21:1179–1183.
Jones T.B., 2001. Liquid dielectrophoresis on the
microscale. J. Electrostatics, 51–52:290–299.
Jones T.B., Gunji M., Washizu M., Feldman M.J., 2001.
Dielectrophoretic liquid actuation and nanoliter
droplet formation. J. Appl. Phys, 89(3):1–8.
Lord Rayleigh, 1879. On capillary phenomena of jets.
Proc. Roy. Soc. (London), 27: 71–97.
Paik P., Pamula V.K., Pollack M.G. and Fair R.B., 2003.
Electrowetting-based droplet mixers for microfluidic
systems. Lab Chip, 3, 28–33.
Park H.Y., Qiu X., Rhoades E., Korlach J., Kwok L.W.,
Zipfel W.R., Webb W.W. and Pollack L., 2006.
Achieving Uniform Mixing in a Microfluidic Device:
Hydrodynamic Focusing Prior to Mixing. J. Anal.
Chem., 78, 4465-4473.
Pellat H., 1895. Mesure de la force agissant sur les
dielectriques liquids non electrises places dans un
champ elitrique. C R Acad Sci, Paris 119:691–694.
Pohl H.A., 1978. Dielectrophoresis, Cambridge University
Press, Cambridge.
Pollack M.G., Shenderov A.D. and Fair R.B., 2002.
Electrowetting-based actuation of droplets for
integrated microfluidics. Lab Chip, 7152:96–101.
Prakash A. R., Amrein M. and Kaler K. V. I. S., 2008.
Characteristics and impact of Taq enzyme adsorption
on surfaces in microfluidic devices. Microfluid
Nanofluid, 4, 295–305.
Prakash R. and Kaler K.V.I.S., 2009. DEP actuation of
emulsion jets and dispensing of sub-nanoliter
emulsion droplets. Lab Chip, Vol. 9:2836–2844.
Singer V.L., Laurie J.J., Yue S.T. and Haugland R.P.,
1997. Characterization of PicoGreen reagent and
development of a fluorescence-based solution assay
for double-stranded DNA quantitation. Anal Biochem,
249:228–238.
Thirukumaran T.K. and Kaler K.V.I.S., 2007. Surface
microfluidics—high-speed DEP liquid actuation on
planar substrates and critical factors in reliable
actuation. J. Micromech Microeng, 17:743–752.
Thorsen T., Maerk S.J. and Quake S.R., 2002.
Microfluidic large-scale integration. Science, 298:580–
584.
Zipper H., Brunner H., Bernhagen J. and Vitzthum F.,
2004. Investigations on DNA intercalation and surface
binding by SYBR Green I, its structure determination
and methodological implications. Nucleic Acids
Research, Vol. 32, No. 12.
BIODEVICES 2010 - International Conference on Biomedical Electronics and Devices
58