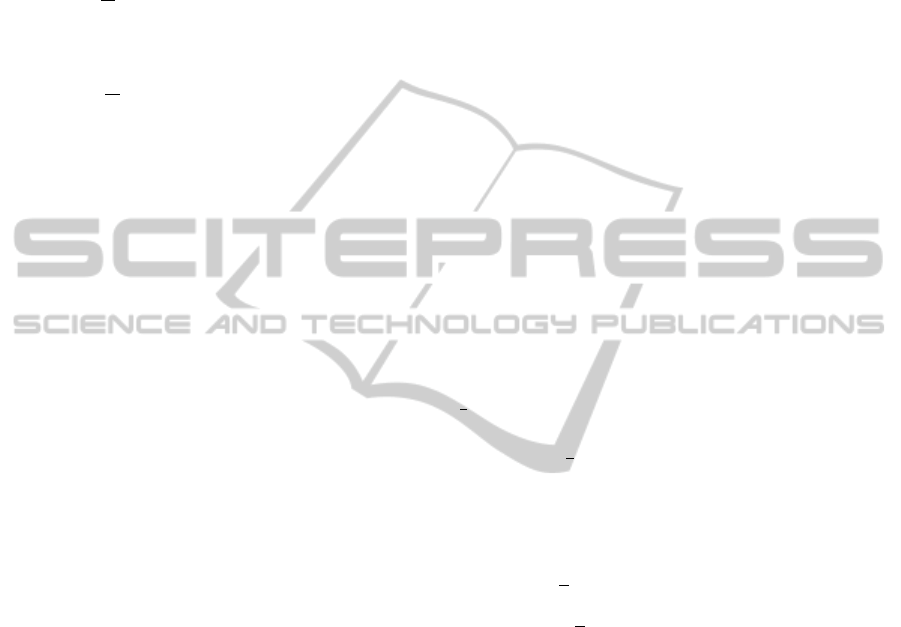
portant in what follows. Consider the quantities
max
k
max
ω≥0
|a
k
(ω)| and of max
k
max
t≥0
|z
k
(t)|. It
turns out that the maxima are achieved for k = N,
which simplifies the notation.
Definition 4.1. We call the system ‘harmonically un-
stable’ if
1
N
lnmax
ω≥0
|a
N
(ω)| > 0 ,
and ‘impulse unstable’ if
1
N
lnmax
t≥0
|z
N
(t)| > 0 .
The system is ‘flock unstable’ if either holds, ‘flock
stable’ otherwise.
Theorem 4.2 ((Veerman et al., 2009), (Veerman,
2010), (Veerman and Tangerman, 2010), (Tanger-
man et al., 2010)). The system given in Definition
1.1 with r = ρ is harmonically and impulse stable
ρ = 1/2 and harmonically and impulse unstable for
ρ ∈ (0,1)\{1/2}.
There are several problems with this. To set up the
definition of flock stability more generally, one would
have to rely on ‘homogeneity’ which is hard to define
(see last paragraph of Section 2). The second problem
is that the Theorem is so hard to prove that it seems
unlikely that substantial generalizations can be made
— at least not using the methods of those papers.
The effect of changing ρ are truly dramatic as the
accompanying figures show. (This was also observed
in (Barooah and Hespanha, 2005).) In Figure 2 (taken
from (Tangerman et al., 2010)) we show a simulation
of the system of Definition 1.1 with r = ρ and with
100 agents, and only slightly vary ρ: 0.45, 0.50, and
0.55. For ρ < 1/2 wild fluctuations of relatively high
frequency occur wiping out every semblance of co-
hesion in short order. For ρ > 1/2 a very large fluc-
tuation occurs over a very large time-scale (a factor
10
3
longer than we can exhibit here). As indicated
before, both systems ultimately stabilize: in practice
this is moot, because the transient fluctuations would
have destroyed cohesion of any actual physical flock
already. In Figure 3 we show (the absolute value of)
the frequency response functions in the three cases:
ρ = 0.45, ρ = 1/2, and rho = 0.55. Note the differ-
ence in scales! When ρ = 1/2 the spectrum the fre-
quency response functions have a very peculiar form
that enables us to deduce the actual dynamics of the
individuals. They undergo a damped stop-and-go mo-
tion (analyzed in (Veerman et al., 2009) and reminis-
cent of post-holiday traffic into urban areas).
These results reveal yet another curious fact. In
finite-dimensional (linear) systems we are used to the
intuition that large fluctuations occur when the eigen-
values associated with the first order ODE (the poles
of a
N
(ω)) get close to the imaginary axis. Here we
vary the dimension N of the system and now this intu-
ition turns out to be completely false! In this case we
can calculate the eigenvalues of the system (see (Da-
Fonseca and Veerman, 2009) and (Tangerman et al.,
2010)). The results are displayed in Figure 4. When
ρ > 1/2 there is an eigenvalue (indicated in red) ex-
tremely close (exponentially in N) to 0; that eigen-
value dominates the behavior of the system. When
ρ = 1/2 there are a couple of eigenvalues roughly at
distance N
−2
of the imaginary axis; these few also
dominate the dynamics. However when ρ < 1/2 all
eigenvalues are bounded away from the imaginary
axis, uniformly in N! Now all eigenvalues contribute
to the dynamics and this gives surprising results: very
large fluctuations. From the point of view of studying
the eigenvalues, this is completely counter-intuitive.
In view of these difficulties we close by encour-
aging (again) the search for a more general point
of view. Let’s go back to the more general sys-
tem given in Proposition 2.1. The physical “work”
W
k
done by the forces acting upon agent k between
time 0 and time t is given by
R
t
0
¨z
k
˙z
k
dt which equals
1
2
(˙z
2
k
(t) − ˙z
2
k
(0)). Employing (·,·), the usual Hermi-
tian inner product on C
N+1
, the sum of the W
k
’s can
be written as
1
2
((˙z, ˙z)(t) − (˙z, ˙z)(0)). After some alge-
bra one obtains for arbitrary Laplacians L
ρ
and L
r
:
Proposition 4.3. The equations given in Proposition
2.1 imply:
1
2
(˙z, ˙z)(t) − f (L
S
ρ
z,z)(t)
=
1
2
(˙z, ˙z)(0) − f (L
S
ρ
z,z)(0)
+g
R
t
0
(L
S
r
˙z, ˙z)dt + f
R
t
0
(L
A
ρ
z, ˙z)dt.
Here for a matrix L we have written L = L
S
+ L
A
,
the latter two denoting the symmetric and the anti-
symmetric parts.
Specialize to the Standard Example. One easily
sees that (˙z, ˙z)(0) = N while (L
S
ρ
z,z)(0) = 0. Perhaps
somewhat surprisingly, the matrices L
S
ρ
and L
S
r
have
one negative eigenvalue (due to the special form of the
equation of motion of the leader). This eigenvalue ap-
pears to have small modulus. Similarly, the matrix L
A
ρ
has non-zero entries only near the ‘boundary-terms’.
Thus the left-hand side tries to be semi-definite posi-
tive while the last two terms of right-hand side is “try”
to be negative, pushing the right-hand side to zero,
but boundary effects slow that down. A more care-
ful analysis would be extremely interesting. The new
aspect is that Proposition 4.3 is valid is completely
SYMMETRY AND STABILITY OF HOMOGENEOUS FLOCKS
473