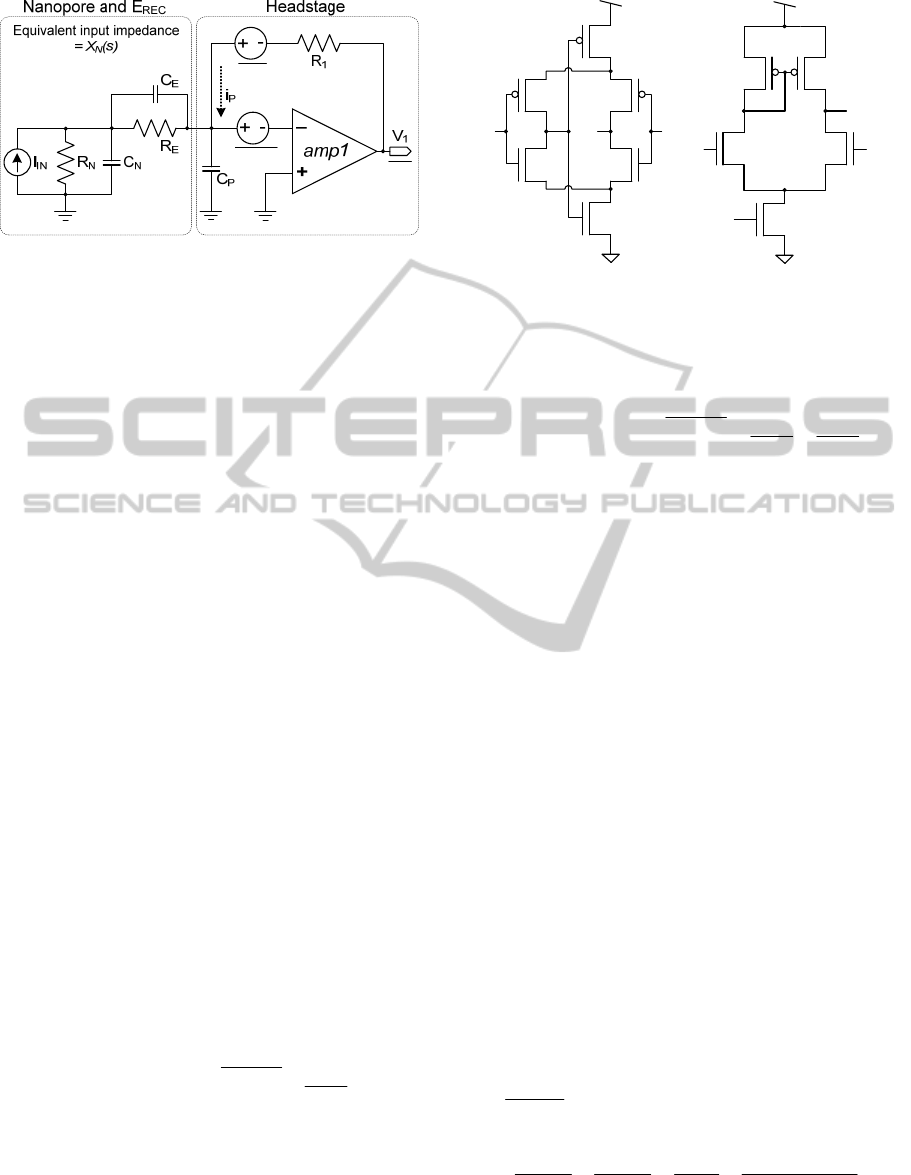
2
,
1
Rn
V
2
1, AMPn
V
2
1,n
V
Figure 4: Simplified electrical model of nanopore, E
REC
and headstage.
Here, k and T are the Botzmann constant and
absolute temperature, respectively. The denominator
of Equation 1, X
N
(ƒ), denotes the impedance of the
equivalent circuit which is expressed in Equation 2.
Because DNA translocation events occur within a
bandwidth of 10KHz and R
E
is negligible compared
to R
N
, X
N
(ƒ) is approximately equal to R
N
at lower
frequencies. Therefore, S
N
(ƒ) is simplified to 4kT/R
N
which is proportional to the conductance of the
nanopore.
In addition to the noise current of the nanopore
and E
REC
, the input noise current of the headstage
has a critical impact on the background noise
because of its input-pair transistor’s 1/ƒ noise, which
increases at lower frequencies. In Figure 4, V
2
n,AMP1
and V
2
n,R1
represent the input-referred noise voltage
of the core-amplifier, amp1, and the thermal noise of
R
1.
The other stages’ noise sources are ignored
because of the relatively high gain of the headstage
(Kim
1
, 2010). Accordingly, the input-referred noise
current of the headstage at lower frequencies is
calculated in Equation 3.
The parameter C
P
is the parasitic capacitance
stemming from a coaxial cable, a BNC connector, a
copper line on PCB, a lead frame, a pad, an ESD cell
and input-pair transistors of the core-amplifier. A(s)
is the amplifier’s transfer function, A
0
/(1+s/ω
0
),
where A
0
and ω
0
represent its open-loop gain and
bandwidth. A(s) can be simplified to A
0
at lower
frequencies. Thus, 1/A(s) in Equation 3 is neglected,
leading to the simplified expression given by
() ( )
1
2
1,
2
4
2
R
kT
VCffS
AMPnPH
+⋅⋅≈
π
(4)
The second term in Equation 4 can be reduced by
increasing R
1
at the cost of larger area and a limited
bandwidth. To diminish the first term, V
2
n,AMP1
also
has to be as low as possible.
V
DD
V
SS
M
1
M
2
M
4
M
3
M
5
M
6
V
IN+
V
IN-
V
OUT
V
DD
V
SS
M
1
M
2
M
4
M
3
V
IN+
V
IN-
V
OUT
M
5
Figure 5: Self-biased differential Bazes amplifier (left) and
general differential amplifier (right).
According to Equations 1 and 4, the background
noise during the nanopore sensing is calculated as
() ( )
N
AMPnPB
R
kT
R
kT
VfCfS
44
2
1
2
1,
2
++⋅≈
π
(5)
This noise equation shows that the thermal noise of
the feedback resistor, 4kT/R
1
, is dominant compared
with the nanopore noise, 4kT/R
N
due to the usual
situation where R
N
>>R
1
. Thus to lessen the overall
background noise, a low-noise core-amplifier should
be designed for the headstage.
3.2 Low-noise Core-amplifier Design
A self-biased differential Bazes amplifier (Bazes,
1991), shown in Figure 5 (left), is used for the low-
noise core-amplifier design in this work. By virtue
of the symmetric structure of NMOS and PMOS
transistors, this amplifier is able to achieve both a
symmetrical positive and negative input common-
mode range (ICMR) and power-supply rejection
ratio (PSRR). Its structure also enables a high gain
because both the NMOS and PMOS contribute to
the effective transconductance (g
m
). Thus, its gain,
(g
m2
+g
m4
)×(r
o3
||r
o5
), where r
o
denotes the transistor
output resistance, is higher than the gain, g
m
×(r
op
||r
on
),
of a differential amplifier, as illustrated in Figure 5
(right), previously used (Weerakoon,2009). Dividing
the output equivalent noise by the squared gain of
the amplifier, the input-referred noise voltage of this
Bazes amplifier is approximately:
()()
()
noiseThermal
mm
noisef
ox
AMPn
gg
kT
fC
K
WLWL
V
2
42
/1
42
2
1,
8211
+
+⋅
⎥
⎦
⎤
⎢
⎣
⎡
+
=
γ
(6)
A FULLY INTEGRATED CMOS SENSOR FOR PICO-CURRENT MEASUREMENT ON SOLID-STATE NANOPORE
DEVICES
29