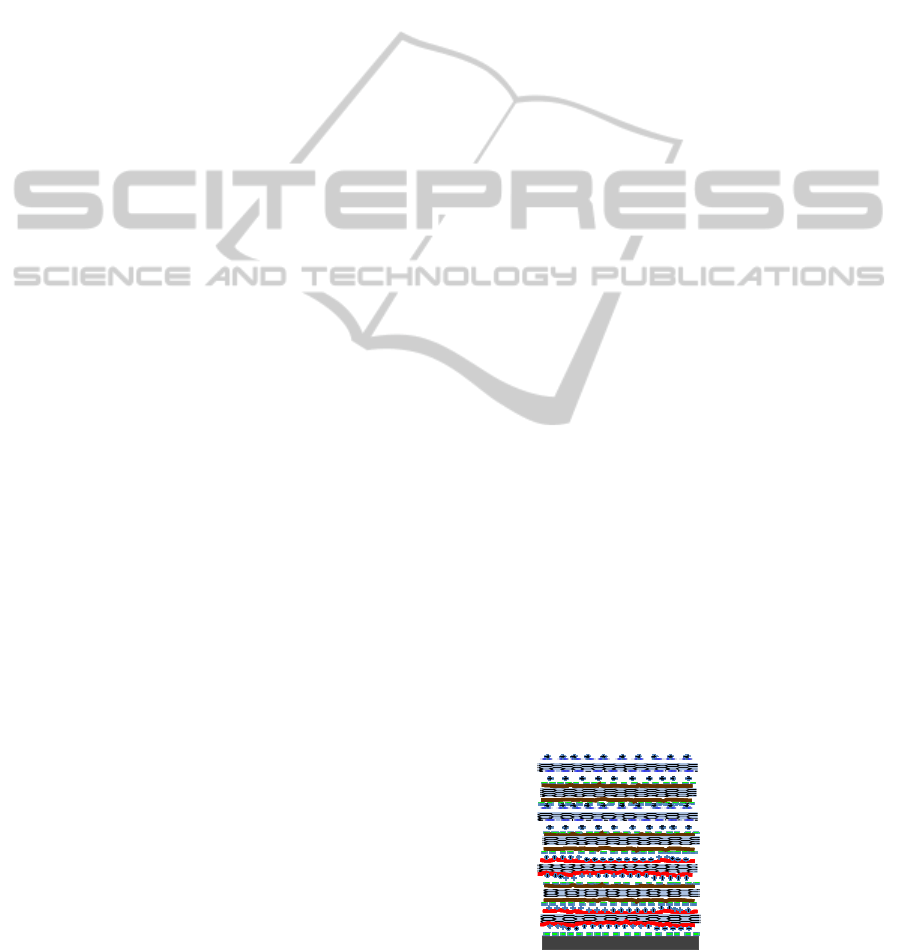
using TGA Q500 (TA Instrument, USA) instrument
in air atmosphere over a temperature range from 30
to 800 °C at a heating rate of 10 °C/min. Raman
spectroscopy was performed using 785 nm laser
excitation (model SDL-8530, SDL Inc.) on
Reinshaw inVia Raman microcope system. FT-IR
measurements were taken for raw, oxidized MWNT
and MWNT-OPH. The samples were ground with
potassium bromide (KBr) to form a very fine
powder using a mortar and pestle. This powder was
then compressed into a thin and transparent pellet
and was placed into the sample holder for analysis.
Analysis was performed using a Shimadzu (Thermo-
Electron Corp., Waltham, MA) bench machine with
32 scans. A drop of MWNT-OPH solution was
placed on the glass slide, allowed to spread
uniformly, and dried over night. The slide was
examined by field emission scanning electron
microscopy equipped with an energy dispersive X-
ray analyzer (JEOL USA, Inc., Peabody, MA).
Cyclic voltammetric and amperometric
measurements were performed using a CV-50
potentiostat (BAS USA) connected to a personal
computer. A three-electrode configuration was
employed, consisting of modified/glassy carbon
(GC) electrode (3-mm diameter) serving as a
working electrode, whereas Ag/AgCl (3 M KCl) and
platinum wire served as the reference and counter
electrodes respectively. Batch electrochemical
experiments were carried out in a 2 ml voltammetric
cell at room temperature (25 °C).
2 LAYER-BY-LAYER ASSEMBLY
OF MWNT THIN FILMS
2.1 Slides
Glass or silicon slides were cleaned in concentrated
H
2
SO
4
/30% H
2
O
2
(3:1). The negatively charged
slides were alternately immersed in aqueous
dispersion of MWNT-PEI and MWNT-DNA. The
adsorption time of 15 min was considered sufficient
for the formation of MWNT monolayer. After each
layer deposition, the substrate was rapidly dried
using 50 psi air from a nozzle for 30 seconds. On top
of these cushioning layers, alternate layers of
MWNT-OPH and MWNT-DNA were deposited by
immersing the slide in aqueous solutions of MWNT-
OPH and MWNT-DNA for 15 min. The surface was
renewed by immersing the slides in MWNT-OPH
solution for 15 minutes. These solutions appear
stable even after a year.
2.2 Glassy Carbon Electrode
The glassy carbon electrode (GCE) was polished
with 0.10 and 0.05 μm alumina slurries and then
ultrasonically cleaned in water for 15 min. The GC
electrode was put into 1 M NaOH solution for 5 min
and potential of 1.2 V was applied to introduce
negative charges on the surface; this was followed
by two washings steps with distilled water. The
positively charged MWNT-PEI was adsorbed by
dipping the negatively charged GC electrode in an
aqueous solution of MWNT-PEI for 15 minutes, and
the MWNT-PEI/GC electrode was dried in nitrogen.
Using the same procedure, a layer of negatively
charged MWNT-DNA was adsorbed. Following
that, MWNT-OPH layer was adsorbed on the
(MWNT-DNA/MWNT-PEI)
4
/GC electrode by
dipping in MWNT-OPH solution, and further
bilayers were formed in the same way. The
modified electrode was stored at refrigerated
conditions until use. All the electrochemical
measurements were performed at room temperature.
A three electrode system containing platinum as
auxiliary electrode, an LbL modified glassy carbon
working electrode and a saturated Ag/AgCl
reference electrode was used. The buffer solution
was 50 mM PBS (pH 7.54). The regeneration of the
biosensor interface was realized by immersing the
sensor in a fresh solution of MWNT-OPH for 15
min.
3 RESULTS AND DISCUSSION
The objective of this work was to design the hybrid
catalytic interfaces based on the interaction of
anionic/cationic biomolecular layers structured with
MWNTs (Figure 1). The initial step requires
assembling of supporting bilayer of oppositely
charged MWNT- polyethyleneimine (PEI) and
MWNT-DNA. This allows for further adsorption of
positively charged complex of MWNT-protein
which adsorbs better on this cushioning support
rather than adsorbing directly on a solid support.
MWNT-PEI (+)
MWNT-DNA (-)
MWNT-DNA (-)
MWNT-PEI (+)
MWNT-DNA (-)
MWNT-OPH (+)
MWNT-OPH (+)
MWNT-PEI (+)
MWNT-DNA (-)
MWNT-DNA (-)
MWNT-PEI (+)
MWNT-DNA (-)
MWNT-OPH (+)
MWNT-OPH (+)
Figure 1: LbL interface design (not in scale): the initial
layers of MWNT-PEI and MWNT-DNA provide support
for subsequent layers of MWNT-OPH and MWNT-DNA.
BIODEVICES 2012 - International Conference on Biomedical Electronics and Devices
128