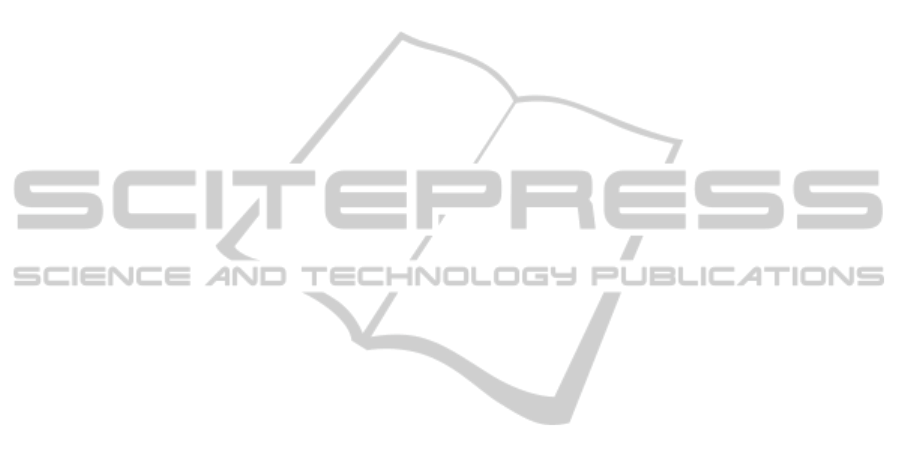
(DAQ) module to the setup, which allows real-time
monitoring. The 3D test layout configuration is
however ideal for device performance. In two-
dimensional configurations long interconnects
between the organic devices and the capacitors result
in excessive energy lost reducing the effectiveness
of PCSs. This low-light demonstration shows the
versatility of the 3D energy harvesting system
configuration. With more techniques (such as vias
and monolithic fabrication) even more energy
efficient 3D configurations can be envisioned and
have been previously reported by our group
(Landrock et al., 2011), however they are not always
convenient for testing purposes.
4 CONCLUSIONS
This work describes an energy harvesting system
comprised of polymer solar cells and a hybrid
polymer energy storage film. We have shown that
this system may be used to generate and store useful
amounts of electrical power, up to several milli-
watts per cm
2
, making it useful for a number of
wireless biomedical sensors applications.
ACKNOWLEDGEMENTS
This research is supported in part by the Natural
Sciences and Engineering Research Council of
Canada (NSERC) and MITACs.
REFERENCES
M. Green et al., “Solar Cell Efficiency Tables (Version
37),” Prog. Photovolt: Res. Appl., vol. 19, 2011,pp.
84-92.
S. H. Park, A. Roy, S. Beaupre, S. Cho, N. Coates, J. S.
Moon, D. Moses, M. Leclerc, K. Lee, A. J. Heeger,
“Bulk Heterojunction Solar Cells with Internal
Quantum Efficiency Approaching 100%”, Nature
Photonics, vol.3, May 2009, pp.297-303.
C. Landrock and B. Kaminska “Ionomer composite thin
film capacitors,” in IEEE Transaction on
Components, Packaging and Manufacturing
Technology, 2nd ed. vol. pp, issue 99, 2011, pp. 1-1.
C. Landrock and B. Kaminska “High temperature polymer
capacitors for aerospace applications,” in Proc.
Design, Autom. Test in Europe (DATE’10), 2010, pp.
1349–1352.
C. Landrock, B. Omrane, Y. Chuo, B. Kaminska, and J.
Aristizabal, “2D and 3D Integration with Organic and
Silicon Electronics,” in Proc. Design, Autom. Test in
Europe (DATE’11), 2011, pp. 1–6
M. Marzencki, K. Toavakolian, Y. Chuo, B. Hung, P. Lin,
B. Kaminska, “Miniature wearable wireless real-time
health and activity monitoring system with optimized
power consumption”, Journal of Medical and
Biological Engineering, 30(4), pp. 227-235.
Z. Xiaodan, X. Xiaoyuan, Y. Libin, L. Yong, “A 1-V 450-
nW fully integrated programmable biomedical sensor
interface chip”, IEEE Journal of Solid-State Circuits,
vol 44, issue 4, pp.1067-1077.
F. C. Krebs, “Air Stable Polymer Photovoltaics Based on a
Process Free From Vacuum Steps and Fullerenes,”
Sol. Energy Mater. Sol. Cells, vol. 92, 2008, pp. 715-
726.
M. Manceau et al., “Further Insights into the
Photodegradation of Poly(3-hexylthiophene) by
Means of X-ray Photoelectron Spectroscopy,” Thin
Solid Films, vol. 518, 2010, pp. 7113-7118.
M. Wang et al., “Performance and Stability Improvement
of P3HT:PCBM-Based Solar Cells by Thermally
Evaporated Chromium Oxide (CrOx) Interfacial
Layer,” ACS Appl. Mater. Interfaces, vol. 2, 2010, pp.
2699-2702.
D. Hohertz, B. Omrane, C. Landrock, Y. Chuo, K.
Kavanagh, B. Kaminska, “Long life indium based
organic photovoltaics,” Procs of SPIE Photonics
North 2011, 4 pages, May 2011.
C. Landrock, “High Temperature Capable Ionic Polymer-
Metal Composite Capacitors and Power Storage
Systems”, Procs. Of SAE Power Systems Congf. Nov.
2010, pp1-17.
J. Aristizabal, B. Omrane, C. Landrock, S. V. Grayli, Y.
Chuo, J. N., Patel, B. Kaminska, C. Menon, “Tungsten
Lamps as an Affordable Light source for Testing of
Photovoltaic Cells”, Journal of Electronic Testing,
vol. 27, no. 3, 2011, pp.403-410.
BIODEVICES 2012 - International Conference on Biomedical Electronics and Devices
244