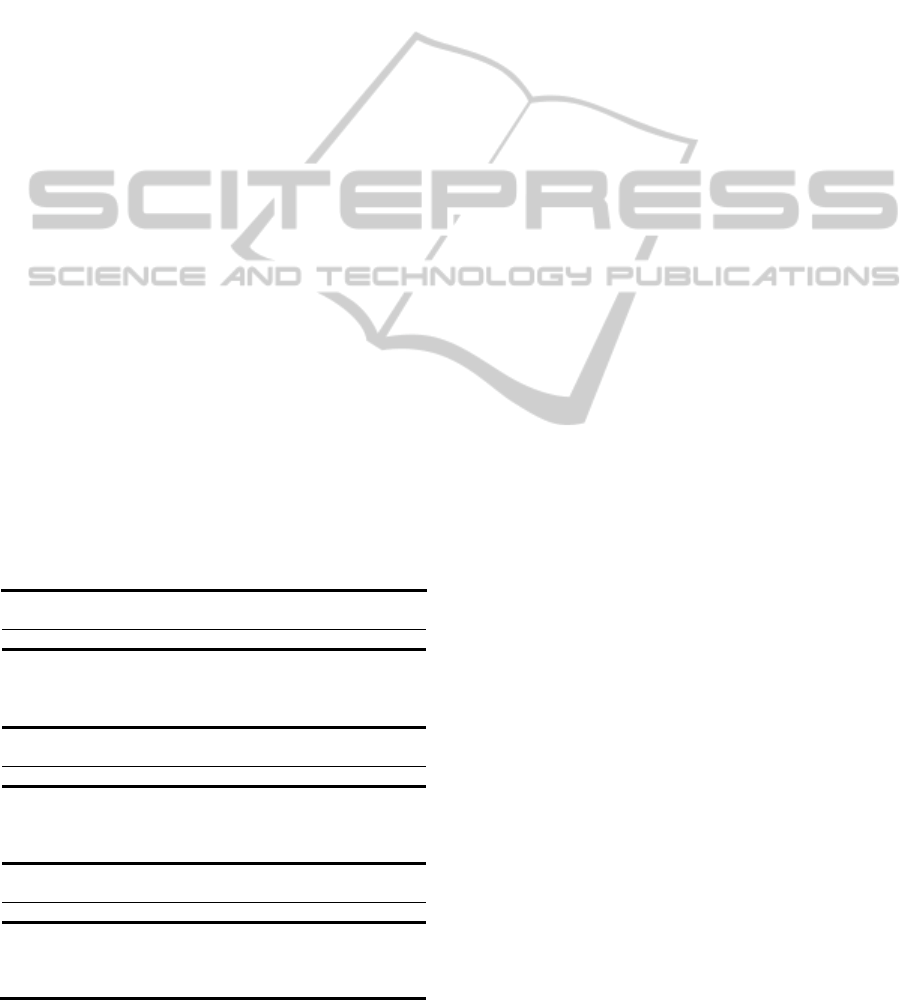
3 RESULTS AND DISCUSSIONS
3.1 Degree of Swelling
The degree of swelling decreased from 8.78 to 6.48
g of water per gram of chitosan-based dried
hydrogel concurrently with the increase in magnetite
concentration from 0.0 to 5.5 wt.-%, respectively.
The degree of swelling of the chitosan-based
magnetic hydrogels was less than that found for the
chitosan-based hydrogel without magnetic behavior
(0 wt.-% of magnetite). The same behavior was
observed with hydrogels based on modified
maltodextrin and modified gum arabic, as a lesser
degree of swelling occurred in magnetic hydrogels.
An electrostatic repulsion generated between the
ionized groups of both the magnetic hydrogels and
the hydrogels without magnetic properties may
expand the polymer network (Jiang et al., 2010).
This phenomenon helps destabilize the structures of
the material, thereby allowing the diffusion of water
and solutes in and out-of the polymer matrix
(Paulino et al., 2009; 2010). Thus, a greater amount
of magnetite used in the hydrogel synthesis leads to
a greater degree of crosslinking due to the formation
of covalent bonds between the iron and hydroxyl or
amine groups. With this logic, a greater density of
crosslinking decreases the swelling rate.
3.2 Water Diffusion Mechanism
Table 1: Water diffusion exponent values (n) at 25
o
C into
hydrogels based on chitosan, modified maltodextrin and
modified gum arabic (pure hydrogel) and with known
amounts of magnetite (magnetic hydrogel).
Chitosan-based hydrogel (Pure hydrogel) and magnetic
hydrogels
(n) (R
2
)
Pure hydrogel
1.9 wt.-% magnetite
0.5478
0.5474
0.9918
0.9907
2.9 wt.-% magnetite 0.5910 0.9841
5.5 wt.-% magnetite 0.6046 0.9885
Modified-maltodextrin-based hydrogel (Pure hydrogel)
and magnetic hydrogels
(n) (R
2
)
Pure hydrogel 0.5430 0.9950
1.9 wt.-% magnetite 0.5545 0.9993
2.9 wt.-% magnetite 0.5479 0.9973
5.5 wt.-% magnetite 0.5492 0.9982
Modified-gum-arabic-based hydrogel (Pure hydrogel) and
magnetic hydrogels
(n) (R
2
)
Pure hydrogel 0.6201 0.9982
1.9 wt.-% magnetite 0.6307 0.9927
2.9 wt.-% magnetite 0.6385 0.9857
5.5 wt.-% magnetite 0.6496 0.9921
Table 1 displays the water diffusion exponent (n) for
hydrogels based on chitosan, modified maltodextrin
and modified gum arabic, containing magnetite
concentrations ranging from 0.0 to 5.5 wt.-%. The n
values ranged from 0.5430 to 0.6496, indicating the
non-Fickian diffusion model, with a tendency
toward macromolecular structure relaxation.
Moreover, these results indicate that there is no
variation in the water diffusion mechanism between
regular and magnetic hydrogels, confirming
previous studies (Paulino et al., 2009; 2010; 2011).
3.3 FTIR Analysis
Fig. 1 displays the FTIR spectra of the chitosan-
based hydrogel and chitosan-based magnetic
hydrogel. All hydrogels exhibited a broad absorption
band between 1715 and 1724 cm
-1
, corresponding to
the carbonyl group stretch in protonated carbonyl
acid, which means that these hydrogels are
polycations. This carbonyl comes from the
acetylated chitosan with a 10% degree of
acetylation. In the chitosan-based magnetic hydrogel
spectrum, the peak shifted to lower values due to
hydrogen bonds and interactions with magnetite
nanoparticles. The band appearing at 1574 cm
-1
corresponds to the amine group vibrations (amide II)
in the chitosan molecule, which interacted with
magnetite nanoparticles, appearing at 1552 cm
-1
in
the magnetic hydrogel spectrum. The band at 1451
cm
-1
may be attributed to the methyl groups. The
peak at 1401 cm
-1
may be associated to CH
2
scissoring of the six carbons of the glucosamine
residues. The sharp bands around 1322 cm
-1
reveal
the presence of carboxylic groups and appeared at
1249 cm
-1
in the magnetic hydrogel spectrum due
the interaction with the magnetite nanoparticles. The
remaining broad absorption bands between 1110 and
1155 cm
-1
may be associated to vibration modes of
the saccharide rings and C-N stretching. The C-O-C
stretching vibration is also expected to take place
around 1000 cm
-1
and appears as a small shoulder on
the broad absorption band. There were important
differences between the chitosan-based hydrogel and
chitosan-based magnetic hydrogel spectra, which
were used to characterize the modification achieved
with the embedding of magnetite nanoparticles. The
main differences were related to the shifting to lower
values of the absorption bands at 1724, 1574 and
1322 cm
-1
due the formation of covalent bonds with
magnetite molecules.
Fig. 2 displays the FTIR spectra of the purified
maltodextrin, glycidyl methacrylate, modified
maltodextrin and modified maltodextrin-based
POLYSSACHARIDE-BASED MAGNETIC HYDROGELS AS POTENTICAL VECTORS FOR
EXTERNAL-CONTROLLED SOLUTE RELEASE
265