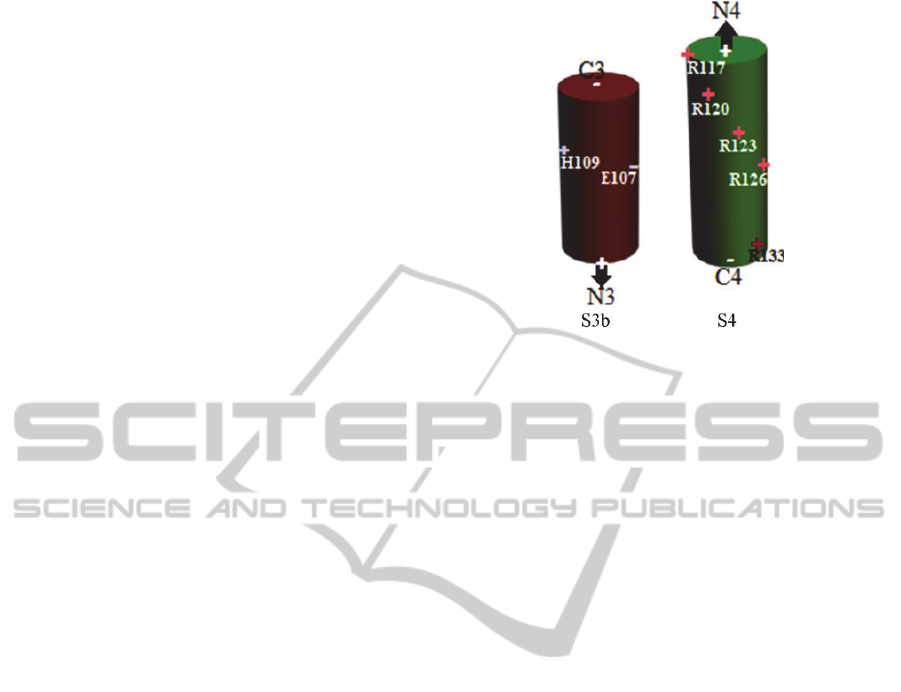
by different methods (Jiang et al., 2003a); (Lee et
al., 2005); (Butterwick and MacKinnon, 2010) the
S3b and orientations. Each of these helices forms a
macrodipole and can potentially contribute to the
electrostatic field.
Here, we have made use of an application of
information technologies and computational
systems to understand the configuration of a pair of
helices (S3b-S4) of the voltage gated potassium ion
channel of Aeropyrum pernix (KvAP) at zero
transmembrane voltage.
2 THEORY
2.1 Model of a Pair of Antiparallel
α-Helix
The voltage-gated ion channel protein (KvAP)
which is a transmembrane helix-turn-helix protein in
lipid and ionic environment is modeled as an
aggregation of macrodipoles in a hybrid or complex
dielectric environment. A α-helix possesses a dipole
moment by virtue of the alignment of its peptide
bonds having half-positive and negative charges at
their ends respectively. For this fractional charge
separation, a single peptide unit behaves like a
microdipole (Wada, 1976). When these microdipoles
align along the axis of the α-helix, making hydrogen
bonds with the neighboring peptide units, it behaves
like a macrodipole (Hol 1978) with positive C-
terminal and negative N-terminal on either end.
The VSD has four such antiparallel α-helix
macrodipoles (S1, S2, S3a-S3b, S4). The S3b-S4
helix-pair “paddle” of the voltage sensor domain
(PDB: 1ORQ) in the x-ray crystallography structure
of KvAP is selected for the simulation of the helix-
pair. The S3b contains a positively charged Histidine
(H109) and a negatively charged Glutamic acid
(E107) (Figure 2). On the other hand S4 contains
five positively charged Arginines (R117, R120,
R123, R126 and R133). Except Histidine which is
half, all the residues have unit charge. Apart from
the charged residues, the contributions of the
terminal charges of the helix dipoles are half unit at
two positive N-terminals (N3, N4) and two negative
C-terminals (C3, C4). The length of the S3b and S4
helices are 19.5Å and 24.0Å respectively. The
Histidine (H109) is 3.0Å above and 200º apart
(clockwise) from E107. On S4 the first charged
residue R117 is at the positive N-terminal (N4),
while the others are placed 60º, 120º, 180º, and 200º
clockwise away from and 4.5Å, 9.0Å, 13.5Å, and
24.0Å below R117 respectively.
Figure 2: The S3b and S4 helix pair with the charges. C3,
N3, N4 and C4 are the terminal dipole charges and the
arrows specify the direction of the dipole moments of the
two helices. R17, R120, R123, R126 and R133 are the
positively charged arginines on S4 and on S3b, H109 and
E107 are positively charged Histidine and negatively
charged glutamic acid respectively. (The figure is
generated by 3D Max).
The interaction potential energy of the system of
charges on S3b-S4 macrodipoles is calculated to
study the mutual configuration between S3b and S4
helices. Three parameters of motion of S4 are
considered. 1) The angle of rotation (θ°) about its
own axis; 2) the relative translational (x Ǻ) with
respect to S3b; 3) the oscillation (°) in the plane
parallel to S3b. At θ=0.0°, x=0.0Å and =0.0°, R117
of S4 helix faces E107 of S3b, which is perfectly
parallel to S4. With the variation of x, S4 slides
along S3b. At different combinations of theta and x
values different residues come in front of E107.
2.2 Electrostatic Principle Holding the
Macrodipoles Together
On the basis of the principle of electrostatic theory,
the antiparallel arrangement is best understood by
dipolar interactions in which the mutual potential
energy (PE) of two interacting adjacent
macrodipoles depends upon their dipole moment ()
and varies with their relative angular separation ()
(Mahajan, 1988). The two dipoles tend to orient so
as to achieve the minimum PE of the system. Lower
the PE, more stable is the conformation. When they
are parallel (=0°) the PE is maximum; when
perpendicular (=90°) PE is zero, and when
antiparallel (=180°), the PE reaches a minimum
value (Mapder and Adhya, 2012).
When the dipoles are very close to each other
(i.e. the distance between the two dipoles is smaller
BIOINFORMATICS2013-InternationalConferenceonBioinformaticsModels,MethodsandAlgorithms
112