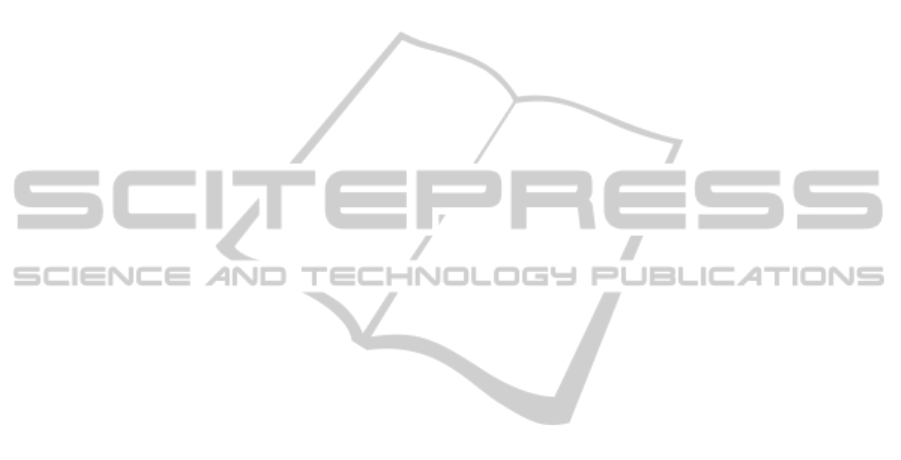
REFERENCES
Buitenweg, J. R., Rutten, W. L. C. and Marani, E., 1999.
‘Finite element modeling of the neuron-electrode
interface’, IEEE Engineering in Medicine and Biology
Magazine, vol.19, no.6, pp. 46-52.
Buitenweg, J. R., Rutten, W. L. C., Marani, E., 2003.
‘Geometry-based finite-element modeling of the
electrical contact between a cultured neuron and a
microelectrode’, Biomedical Engineering, IEEE
Transactions on, vol.50, no.4, pp.501-509.
Choi, C. T. M., You, S., 2012. ‘Finite element models of
neuron electrode sealing interfaces’, Magnetics, IEEE
Transactions on, vol.48, no.2, pp.643-646.
Cogan S. F., 2008. ‘Neural stimulation and recording
electrodes’, Ann. Rev. Biomed. Eng, vol.10, pp.275-
309.
Elia S., Lamberti P., Tucci V., 2009. ‘A finite element
model for the axon of nervous cells’, COMSOL
Conference.
Huang, X., Nguyen, D., Greve, D. W., Domach, M. M.,
2004. ‘Simulation of microelectrode impedance
changes due to cell growth’, Sensors Journal, IEEE,
vol.4, no.5, pp. 576- 583.
Lu T. Z., Feng Z-P, 2011. ‘A sodium leak current
regulates pacemaker activity of adult central pattern
generator neurons in Lymnaea Stagnalis’. PLoS ONE,
vol.6, no.4, p.e18745.
McCreery, D. B., Agnew, W. F., Yuen, T. G., Bullara, L.,
1990. ‘Charge density and charge per phase as
cofactors in neural injury induced by electrical
stimulation’. IEEE Trans. Biomed. Eng., vol.37, no.10,
pp.996–1001.
Molleman A., 2003. ‘Basic Theoretical Principles, in
Patch Clamping: An Introductory Guide To Patch
Clamp Electrophysiology’, John Wiley & Sons, Ltd,
Chichester, UK.ch2.
Moulin C., Gliere A., Barbier D., Joucla S., Yvert B.,
Mailley P., Guillemaud R., 2008. ’A new 3-D finite-
element model based on thin-film approximation for
microelectrode array recording of extracellular action
potential’ IEEE Trans. Biomed. Eng., Vol.55, pp.683-
92.
Rutten, W. L. C. 2002. Annu. Rev. Biomed. Eng. 4, 407.
Schoen I, Fromherz P., 2007. ‘The mechanism of
extracellular stimulation of nerve cells on an
electrolyte-oxide-semiconductor capacitor’. Biophys.
J., 92, vol.92, no.3, pp.1096–1111.
Schoen, I., Fromherz, P., 2008. ‘Extracellular stimulation
of mammalian neurons through repetitive activation of
Na+ channels by weak capacitive currents on a silicon
chip’. J Neurophysiol, vol.100, no.1, pp.346–357
Syed, N. I., Zaidi, H., Lovell, P., 1999. U. Windhorst, H.
Johansson (Eds.),’ Modern Techniques in
Neuroscience Research’, Springer, Berlin, Heidelberg
, pp. 361–377.
Wei X. F., Grill W. M., 2009. ‘Analysis of high-perimeter
planar electrodes for efficient neural stimulation’.
Front. Neuroeng. , vol.2, no.15.
Yúfera, A., Olmo, Daza, P and Cañete, D. A., 2003. ‘Basic
Theoretical Principles, in Patch Clamping: An
Introductory Guide To Patch Clamp
Electrophysiology’, John Wiley & Sons, Ltd,
Chichester, UK. ch2.
BIODEVICES2013-InternationalConferenceonBiomedicalElectronicsandDevices
56