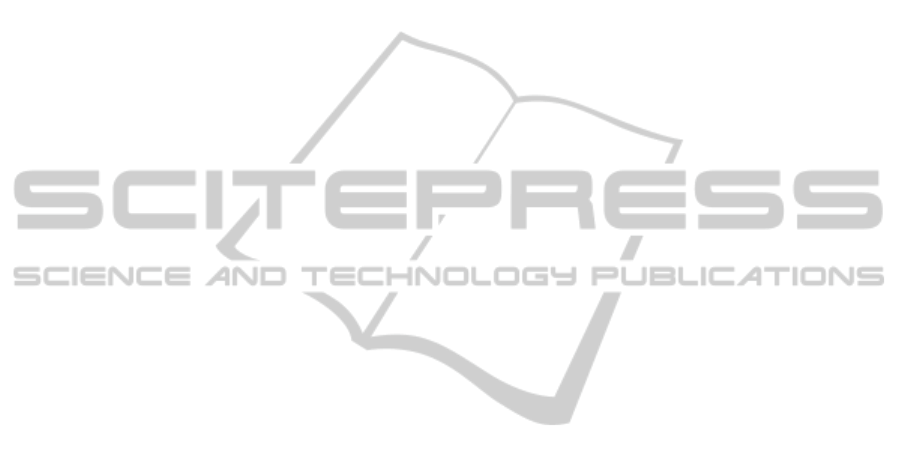
REFERENCES
Bootsma, R. J. & Van Wieringen, P. C., 1990. Timing an
attacking forehand drive in table tennis. Journal of
Experimental Psychology: Human Perception and
Performance, 16(1), pp.21–29.
Carlsen, A. N. et al., 2011. Considerations for the use of a
startling acoustic stimulus in studies of motor
preparation in humans. Neuroscience and
biobehavioral reviews, 35(3), pp.366–76.
Carlsen, A. N. et al., 2008. Motor preparation in an
anticipation-timing task. Experimental brain research,
190(4), pp.453–61.
Carlsen, A. N., Chua, R. & Inglis, J., 2004. Can prepared
responses be stored subcortically? Experimental brain
research, 159(3), pp.301–9.
Carlsen, A. N. & Mackinnon, C. D., 2010. Motor
preparation is modulated by the resolution of the
response timing information. Brain research, 1322,
pp.38–49.
Cooke, J. D. & Diggles, V. A., 1984. Rapid error
correction during human arm movements: evidence for
central monitoring. Journal of motor behavior, 16(4),
pp.348–63.
Desmurget, M. & Grafton, S., 2000. Forward modeling
allows feedback control for fast reaching movements.
Trends in cognitive sciences, 4(11), pp.423–431.
Floeter, M. K. & Rothwell, J. C., 1999. Releasing the
brakes before pressing the gas pedal. Neurology, 53(4),
pp.664–664.
Gray, R., 2002a. Behavior of college baseball players in a
virtual batting task. Journal of Experimental
Psychology: Human Perception and Performance,
28(5), pp.1131–1148.
Gray, R., 2002b. “Markov at the Bat”: A Model of
Cognitive Processing in Baseball Batters.
Psychological Science, 13(6), pp.542–547.
Higgins, J. R. & Angel, R. W., 1970. Correction of
tracking errors without sensory feedback. Journal of
experimental psychology, 84(3), pp.412–6.
Marinovic, W., Plooy, A. M. & Tresilian, J. R., 2009. The
utilisation of visual information in the control of rapid
interceptive actions. Experimental psychology, 56(4),
pp.265–73.
Maslovat, D., Carlsen, A. N. & Franks, I. M., 2012.
Subcortical motor circuit excitability during simple
and choice reaction time. Behavioral neuroscience,
126(3), pp.499–503.
McMillan, S., Nougier, V. & Byblow, W., 2004. Human
corticospinal excitability during a precued reaction
time paradigm. Experimental brain research, 156(1),
pp.80–7.
Müller, S. & Abernethy, B., 2012. Expert anticipatory skill
in striking sports: a review and a model. Research
quarterly for exercise and sport, 83(2), pp.175–87.
Nakamoto, H. & Mori, S., 2012. Experts in fast-ball sports
reduce anticipation timing cost by developing
inhibitory control. Brain and cognition, 80(1), pp.23–
32.
Regan, D., 1992. Visual judgements and misjudgements in
cricket, and the art of flight. Perception, 21(1), pp.91–
115.
Reynolds, C. & Ashby, P., 1999. Inhibition in the human
motor cortex is reduced just before a voluntary
contraction. Neurology, 53(4), pp.730–5.
Runigo, C. Le, Benguigui, N. & Bardy, B. G., 2005.
Perception-action coupling and expertise in
interceptive actions. Human movement science, 24(3),
pp.429–45.
Runigo, C. Le, Benguigui, N. & Bardy, B. G., 2010.
Visuo-motor delay, information-movement coupling,
and expertise in ball sports. Journal of sports sciences,
28(3), pp.327–37.
Soto, O., Valls-Solé, J. & Kumru, H., 2010. Paired-pulse
transcranial magnetic stimulation during preparation
for simple and choice reaction time tasks. Journal of
neurophysiology, pp.1392–1400.
Starr, A. et al., 1988. Enhancement of motor cortical
excitability in humans by non-invasive electrical
stimulation appears prior to voluntary movement.
Electroencephalography and clinical neurophysiology,
70(1), pp.26–32.
Valls-Solé, J. et al., 1999. Patterned ballistic movements
triggered by a startle in healthy humans. The Journal
of physiology, 516, pp.931–8.
Wolpert, D. M., Ghahramani, Z. & Jordan, M., 1995. An
internal model for sensorimotor integration. Science,
269(5232), pp.1880–1882.
icSPORTS2013-InternationalCongressonSportsScienceResearchandTechnologySupport
12