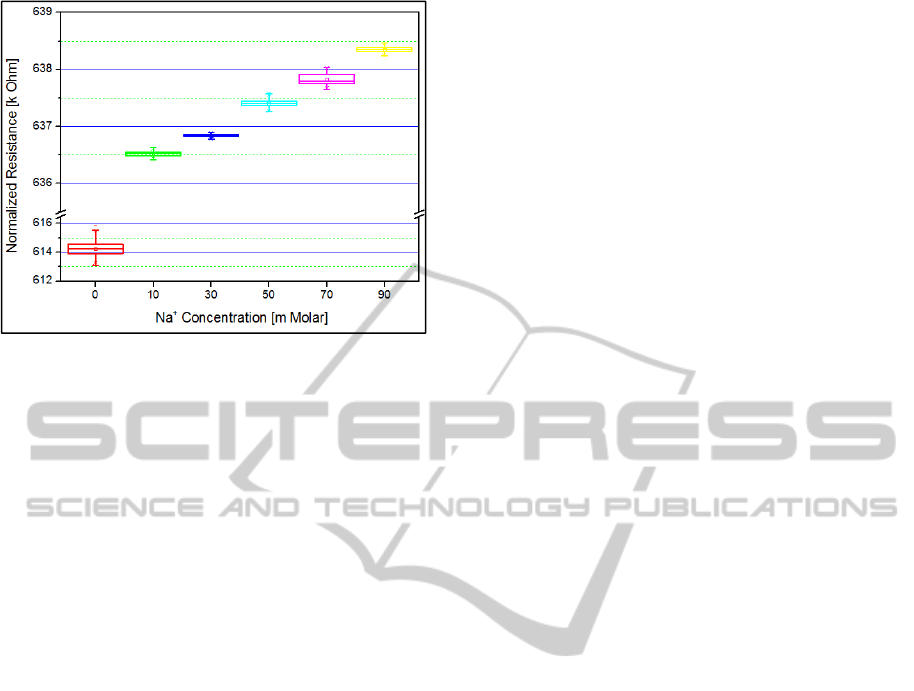
Figure 4: Normalized electrical resistance output vs.
sodium ion concentration input.
(such as the forearm, chest and scapula) were
consistently higher (mean Na+ concentration at each
site: 42.2±25.8, 47.6±25.7 and 42.2±24.8 mM
respectively). Therefore, our sensor operates within
the typical physiological range. As can be seen from
the large standard deviations for sweat Na
+
levels,
sweat composition can vary widely between
individuals. Our sensor is therefore of interest in the
field of exercise science: real-time monitoring of
sweat Na
+
losses allows for the development of
athlete-specific rehydration strategies.
In theory, the lower detection window of the
reported transducer is only limited to the sodium
ionophore applied and electronic amplification
equipment used, as graphene had already been
shown to detect 1 single molecular binding events
(Schedin et al., 2007). As a comparison, a single liter
of 10mM of sodium chloride solution contains
6.023x10
26
number of sodium ions. With such high
sensitivity, this RGO-based sensor can also be
modified with antibodies or other ionophores to
detect other specific ions or biomolecules of interest
to the community. The presented sodium sensor
could potentially be used to diagnose dehydration in
the ageing population and used in the study of the
biology of sweat in older people. Future work on the
disposable and low-cost real-time fully-organic
sensor platform would include optimizing the
fabrication technique for mass-production and
sensing for other ions or proteins.
3 CONCLUSIONS
During exercise, one of the body’s major ionic
deficits of concern is Na
+
. Measurements of sodium
ions in sweat had been reported previously to predict
changes in serum. In this paper, we have shown the
fabrication, characterisation and physiologically-
relevant sodium-ions detection limits of a novel
RGO-based sensor with an integrated pump that is
fully organic, low-cost and disposable. As the sensor
is small, light and wearable, it has enormous
potential to be integrated onto the human body
during field training to obtain real-time data for
immediate intervention.
ACKNOWLEDGEMENTS
The research was supported by the National Institute
for Health Research (NIHR) Diet, Lifestyle &
Physical Activity Biomedical Research Unit based at
University Hospitals of Leicester and Loughborough
University. The views expressed are those of the
authors and not necessarily those of the NHS, the
NIHR or the Department of Health. The research
was also funded by the Institute for Sports Research
(ISR) of Nanyang Technological University (NTU).
REFERENCES
Baker, L. B., Stofan, J. R., Hamilton, A. A. & Horswill, C.
A., 2009. Comparison of regional patch collection vs.
whole body washdown for measuring sweat sodium
and potassium loss during exercise. Journal of Applied
Physiology, 107, 887-895.
Costill, D. L., 1977. Sweating: Its Composition and
Effects in Body Fluids. Annals of the New York
Academy of Sciences, 301, 160-174.
Coyle, S., King-Tong, L., Moyna, N., O'gorman, D.,
Diamond, D., Di Francesco, F., Costanzo, D., Salvo,
P., Trivella, M. G., De Rossi, D. E., Taccini, N.,
Paradiso, R., Porchet, J. A., Ridolfi, A., Luprano, J.,
Chuzel, C., Lanier, T., Revol-Cavalier, F.,
Schoumacker, S., Mourier, V., Chartier, I., Convert,
R., De-Moncuit, H. & Bini, C., 2010. Biosensing
Textiles for Personalised Healthcare Management.
Information Technology in Biomedicine, IEEE
Transactions on, 14, 364-370.
Gia, 2012. Biosensors in Medical Diagnostics. Global
strategic business report. CA.
He, Q., Sudibya, H. G., Yin, Z., Wu, S., Li, H., Boey, F.,
Huang, W., Chen, P. & Zhang, H., 2010. Centimeter-
Long and Large-Scale Micropatterns of Reduced
Graphene Oxide Films: Fabrication and Sensing
Applications. ACS Nano, 4, 3201-3208.
Huang, J., Harvey, J., Chen, H., Fam, W. H. D., Nimmo,
M. A. & Tok, I. Y. A., 2013a. Growth of Graphene
Oxide Using Ethanol CVD. Procedia Engineering.
Huang, J., Harvey, J., Fam, W. H. D., Nimmo, M. A. &
FullyOrganicGrapheneOxide-basedSensorwithIntegratedPumpforSodiumDetection
87