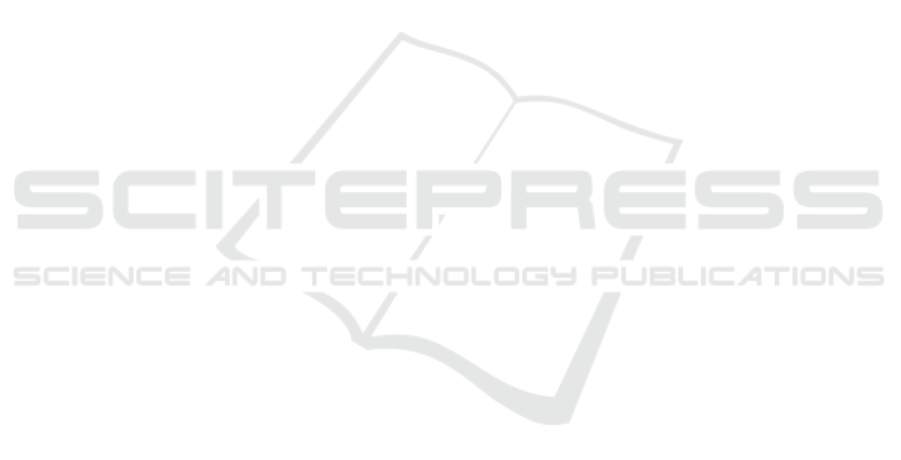
Molding Microchannel and Brain Implant Scaffolds from
Microstructured Double Layer Photo Resin Master Casts
Concepts and Examples
Rouhollah Habibey, Asiyeh Golabchi and Axel Blau
Fondazione Istituto Italiano di Tecnologia (IIT), Dept. of Neuroscience and Brain Technologies (NBT),
Neurotechnologies (NT), Via Morego 30, 16163 Genoa, Italy
1 OBJECTIVES
Replica-casting finds wide application in soft
lithography (Qin et al., 2010) and microfluidics
(Bettinger et al., 2010). Most commonly, structures
are molded with micro- to nano-patterned
photoresists as master casts into
polydimethylsiloxane (PDMS). PDMS features
many favorable properties. It reproduces geometric
details with nanometer fidelity, has low cytotoxicity,
is transparent in the visible spectrum, biostable both
in vitro and in vivo, can be plasma-bonded to itself,
has low water permeability and is simple to handle
and process. After curing, the PDMS can be easily
peeled from the master and the latter usually be
reused (Anderson et al., 2000) if patterns are not
undercut (Yun et al., 2008). Here, we demonstrate a
straightforward replica-molding process that can be
exploited for the generation of perforated
microchannel scaffolds for the in vitro use in axonal
guidance and regeneration studies on microelectrode
arrays (MEAs) or the production of tissue-conformal
in vivo MEAs for neuroprosthetic applications,
respectively.
2 METHODS
Bi-level casting patterns in high-aspect ratio
negative photoresist (e.g., SU-8) to generate
microchannels (lower level) and perforating vias
(second level) are made by standard
photolithography. A first mask defines all features, a
second mask just the through holes. Figure 1
summarizes the following fabrication steps: (1) A
clean silicon wafer is spin-coated (2) with a 1
st
negative photo resin layer (<50 µm) and (3) UV
photo-crosslinked through a first photomask to
define both channels and sockets for vias. The
procedure is repeated in (4) and (5) for a 2
nd
photoresist layer (< 150 µm) to define the via
through-holes. After removing uncured photoresin
(6), the bi-level microstructure (7) can be coated
with PDMS pre-polymer (8), cured after its leveling,
and peeled to result in a microchannel scaffold with
via holes (9).
Such microchannels can either be used as
physical guidance cues for axons and dendrites or be
filled with conductive polymers (e.g., PEDOT:PSS,
carbon-polymer composites) (10) and backside-
insulated (11) to yield MEAs with electrodes and
contact pads at the via holes for in vitro and in vivo
application (12).
3 RESULTS
Figure 2 depicts a conceptual cartoon of a
microchannel tile with via holes for cell seeding
(somata) and guidance channels for axonal
elongation into target wells (axons). If the substrate
is a MEA, electrodes can extracellularly record
action potentials (Figure 3). This allows for studying
neural development and axonal regeneration e.g.,
after inflicting injury by laser microdissection
(Difato et al., 2011).
Furthermore, micro-channels will help in gaining
a better understanding of the electrode
characteristics and underlying biophysics of signal
generation and spread.
If instead microchannels and through-holes are
filled with a biocompatible electrical conductor,
MEAs can be inexpensively produced for both in
vitro and in vivo application (Figure 4).
The flexibility of PDMS allows for the
fabrication of highly tissue-conformal devices with
softness that matches that of brain tissue. This
promises the reduction of implant-inflicted tissue
damage and the increase of MEA long-term
stability.
Habibey R., Golabchi A. and Blau A..
Molding Microchannel and Brain Implant Scaffolds from Microstructured Double Layer Photo Resin Master Casts - Concepts and Examples.
Copyright
c
2013 SCITEPRESS (Science and Technology Publications, Lda.)