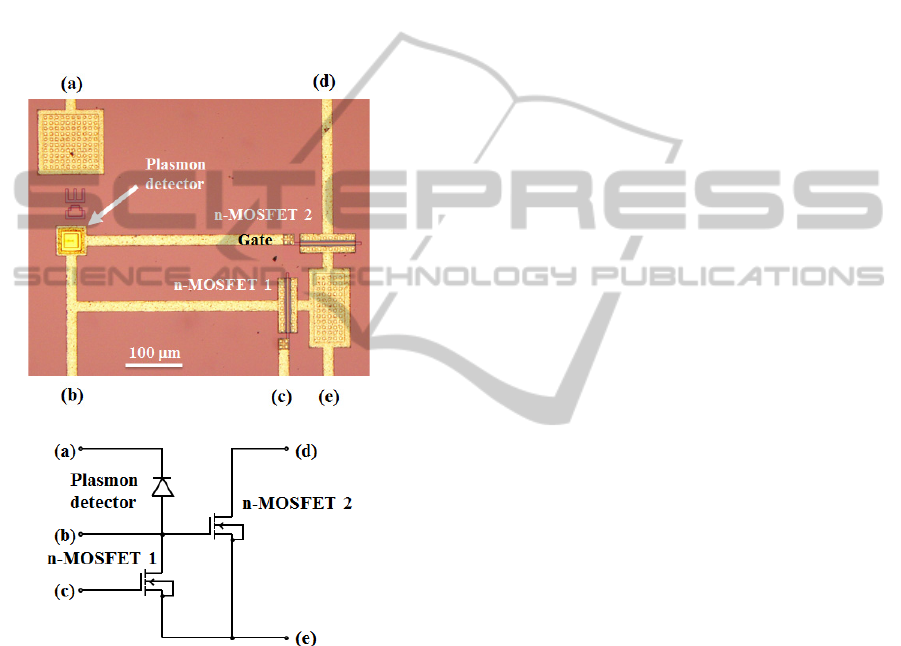
3 DEVICE INTEGRATION
Based on the architecture discussed in section 2, a
part of the devices developed was monolithically
integrated with electronic devices onto silicon
substrates. One example is shown in Figure 7
(Aihara et al., 2013). The surface plasmon (or light)
detector is set on the gate electrode of a MOSFET.
This surface plasmon detector and two MOSFETs
IC operated well under DC- and AC-bias, and a
photocurrent converted from incident light through
surface plasmons was electrically amplified.
(a) optical micrograph of the top view.
(b) circuit diagram.
Figure 7: An integrated circuit of a surface plasmon
detector and two MOSFETs.
4 CONCLUSIONS
Using architectures for surface plasmon devices
developed in our group, some suitable structures of
surface plasmon devices have been discussed for
monolithic integration with electronic devices on a
silicon substrate. In the devices, surface plasmons
transparent to silicon were used to prevent
absorption by silicon, and the use of transparent light
with metal waveguides enables easy control of these
surface plasmons. These techniques allow
integration of surface plasmon devices with
electronic devices using standard CMOS process.
The device integration opens a new phase for
nanoscale surface plasmon ICs.
ACKNOWLEDGEMENTS
This study was partially supported by JSPS
KAKENHI Grant Numbers 22360142, 25630147.
REFERENCES
Aihara, T, Nakagawa, K. Fukuhara, M, Yu, Y. L,
Yamaguchi, K, Fukuda, M. (2011) Optical frequency
signal detection through surface plsmon polaritons.
Appl. Phys. Lett. 99, 043111-1-043000-3.
Aihara, T, Fukuda. M. (2012) Observation of plasmonic
frequency-modulated signal transmission. In IEEE
Optical MEMS & Nanophotonics 2012 (65) Alberta,
Canada. IEEE.
Aihara, T, Fukuda. M. (2012) Transmission properties of
surface-plsmon-polariton coherence. Appl. Phys. Lett.
100, 213115-1-213115-4.
Aihara, T, Fukuhara, M, Takeda, A, Futagawa, M, ¿??,
Sawada, K. Fukuda, M. (2013) Monolithic Integration
of surface plasmon detector and metal-oxide-
semiconductor field-effect transistors. IEEE Photonics
J. 5, 6800609.
Akbari, A, Tait, R. N, Berini, P. (2010) Surface plasmon
waveguide Schottky detector. Opt. Express 18, 8505-
8514.
Barnes, W. L, Dereux, A, Ebbesen, T. W. (2003) Surface
plasmon subwavelength optics. Nature 424, 824–830.
Boltasseva, A, Nikolajsen, T, Leosson, K, Kjaer, K,
Larsen, M. S, Bozhevolnyi, S. I. (2005) Integrated
optical components utilizing long-range surface
plasmon polaritons. J. Lightwave Technol. 23, 413-
422.
Sergey, I, Bozhevolnyi, Valentyn, S, Volkov, Devaux, E,
Laluet, J. –Y, Ebbesen, T. W. (2006) Channel
Plasmon subwavelength waveguide components
including interferometers and ring resonators. Nature
440, 508-511.
Casalino, M, Sirleto, L, Lodice, M, Saffioti, N, Gioffre, M,
Rendina, I, Coppola, G. (2010) Cu/p-Si Schottky
barrier-based near infrared photodetector integrated
with a silicon-on-insulator waveguide. Appl. Phys.
Lett. 96, 241112-1-241112-3.
Ebbesen, T. W, Genet, C, Boshevolnyi, S. I. (2008)
Surface Plasmon circuitry. Phys. Today, May, 44-50.
Fukuda, M, Yamasaki, Y, Oota, N, Utsumi, A. (2007)
Optical near-field generation and detection by using
metal-dielectric film fabricated from silver paste and
spherical fused silica. IEEE Photon. Technol. Lett. 19,
1160-1162.
PHOTOPTICS2014-InternationalConferenceonPhotonics,OpticsandLaserTechnology
156