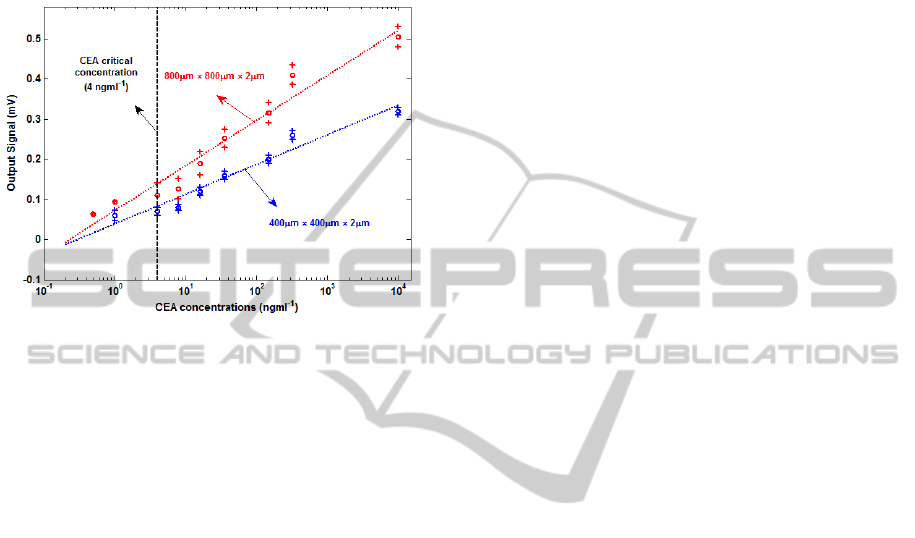
stabilized membrane. The liquid temperature was
precisely controlled and external noise sources were
excluded using a shield box. In order to estimate the
nonspecific adsorption on the MM surface, the
concentration of HSA in all solutions was stabilized
at 0.1 mg/ml.
Figure 3: Steady-state output signals (V
out
) as a function of
CEA concentrations for two different MM geometries.
Every data point on this plot represents an average of
output signals obtained in multiple experiments done with
different MM, whereas the range of output signals
obtained from these experiments is shown as the error bar.
Figure 3 shows the steady-state output signals
(V
out
) as a function of CEA concentration in a HSA
background for different dimensions of MM. By
using a 400 µm x 400 µm x 2 µm MM, the lowest
CEA concentration that we could clearly detect
above noise was 1 ng/ml. However, when a 800 µm
x 800 µm x 2 µm MM was used, CEA concentration
as low as 0.5 ng/ml was detectable. This is close to
the resolution required for CEA-based diagnosis of
prostate cancer (Brian et al., 2011). The
experimental results presented a range of linearity of
0.5 ng/ mL to 10 µg/mL and 1 ng/mL to 10 µg/mL
for 800 µm x 800 µm x 2 µm and 400 µm x 400 µm
x 2 µm MM, respectively. The minimum detectable
surface stress for each sensor can be obtain when the
output signals are equal to the noise values. By using
the experimental results, 2 and 3.5 mJ/m were
respectively the minimum surface stress sensitivities
for the 800 µm x 800 µm x 2 µm and 400 µm x 400
µm x 2 µm MM.
5 CONCLUSIONS
We have reported a novel signal transduction
biosensor for detecting CEA, using a unique micro-
fabricated self-sensing array of MM sensors. Unlike
cantilever sensors, which are based on optical
readout systems, the MM integrated piezoresistive
readout sensors facilitate the detection of compact
devices in even non-transparent environments. our
unique MM design significantly improves sensor
sensitivity that allows us to detect CEA
concentrations as low as 500 pg/ mL, or 3 pM.
REFERENCES
Aeschimann L., Meister A., Akiyama T., Chui B. W.,
Niedermann P., Heinzelmann H., De Rooij N. F.,
Staufer U. and Vettiger P., Microelectron. Eng., 83
(2006) 1698.
Alvarez M. and Lechuga L. M., Analyst, 135 (2010) 827.
Arlett, J. L., Maloney, J. R., Gudlewski, B., Muluneh, M.,
Roukes, M. L. Nano Lett., 6 (2006) 10 00.
Arlett J. L., Myers E. B. and Roukes M. L., Nat.
Nanotechnol., 6 (2011) 203.
Berger R., Delamarche E., Lang H. P., Gerber C.,
Gimezewski J. K., Meyer E. and Guntherodt, H.-J.,
Science, 276 (1997) 2021.
Boisen A., Dohn S., Keller S. S., Schmid S. and Tenje M.,
Rep. Prog. Phys., 74 (2011) 036101.
Boisen A. and Thundat T., Mater. Today, 12 (2009) 32.
Brian B., Shaker A M., Nanotech. Sci. Applic, 4 (2011) 1.
Ghatkesar M. K., Lang H. P., Gerber C., Hegner M. and
Braun T., PLoS One, 3 (2008) 3610.
Goericke F. T. and King W. P., IEEE Sens. J., 8 (2008)
1404.
Guntherodt H. J., Anal. Chim. Acta 393 (1999) 59.
Harley, J.A.and Kenny, T.W., J. Microelectromech. Syst.,
9 (2000) 226.
Hooge, F. N., Phys. Lett. A, 29 (1969) 139.
Lang H. P., Baller M. K., Berger R., Gerber C.,
Gimzewski J. K., Battiston F. M., Fornaro P.,
Ramseyer J. P., Meyer E. And Guntherodt H. J., Anal.
Chim. Acta 393 (1999) 59.
Loui A., Goericke F. T., Ratto T. V., Lee J., Hart B. R.
and King W. P., Sens. Actuators, A, 147 (2008) 516.
Mukhopadhyay R., Sumbayev V. V., Lorentzen M.,
Kjems J., Andreasen P. A. and Besenbacher F., Nano
Lett., 5 (2005) 2385.
Noelia D., Paula D., Sergio M., María G., Sara P., Alberto
O. and Manuel F., Sens., 12 (2012) 2284.
Omidi M., Malakoutian M. A., Choolaei M., Chin. Phys.
Lett., 30(6) (2013) 068701.
Privorotskaya N. L. and King W. P., Microsyst. Technol.,
15 (2008) 333.
Tufte O. N., and E. L. Stelzer, J. Appl. Phys., 34 (1963)
313.
Wu G., Datar R. H., Hansen K. M., Thundat T., Cote R. J.
and Majumdar A., Nat. Biotechnol. 19 (2001) 856.
Yang, S. M., Yin, T. I. and Chang, C. Sens. Actuators. B,
121 (2007) 545.
BIODEVICES2014-InternationalConferenceonBiomedicalElectronicsandDevices
138