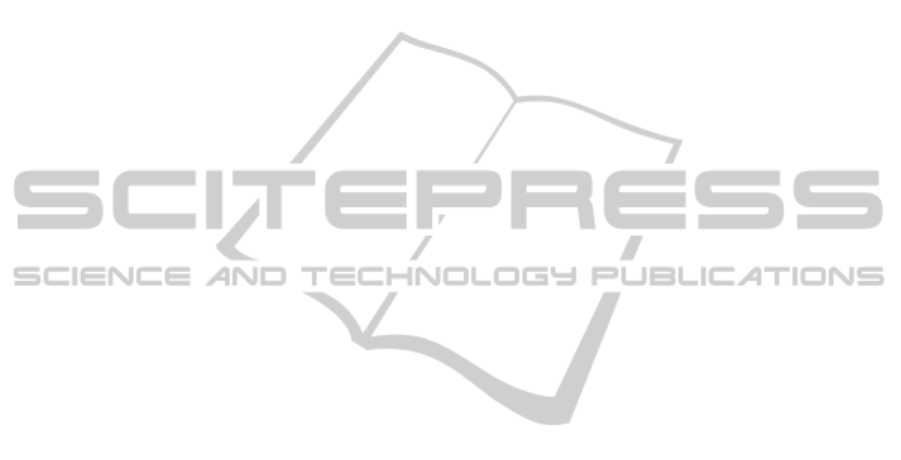
Giannotti, S., Bottai, V., DellOsso, G., Pini, E., De Paola,
G., Bugelli, G., and Guido, G. (2013). Current
medical treatment strategies concerning fracture heal-
ing. Clinical Cases in Mineral and Bone Metabolism,
10(2):116.
Giannoudis, P. V., Einhorn, T. A., and Marsh, D. (2007).
Fracture healing: the diamond concept. Injury, 38:S3–
S6.
Goldstein, C., Sprague, S., and Petrisor, B. A. (2010). Elec-
trical stimulation for fracture healing: current evi-
dence. Journal of Orthopaedic Trauma, 24:S62–S65.
Goodship, A. E., Lawes, T. J., and Rubin, C. T. (2009).
Low-magnitude high-frequency mechanical signals
accelerate and augment endochondral bone repair:
Preliminary evidence of efficacy. Journal of Or-
thopaedic Research, 27(7):922–930.
Griffin, M. and Bayat, A. (2011). Electrical stimulation in
bone healing: critical analysis by evaluating levels of
evidence. Eplasty, 11:303–353.
Hak, D. J., Toker, S., Yi, C., and Toreson, J. (2010). The
influence of fracture fixation biomechanics on fracture
healing. Orthopedics, 33(10):752–755.
Heckman, J. D. and Sarasohn-Kahn, J. (1997). The eco-
nomics of treating tibia fractures. Bulletin Hospital
for Joint Diseases, 56(1):63–72.
Huang, R. P., Rubin, C. T., and McLeod, K. J. (1999).
Changes in postural muscle dynamics as a function of
age. The Journals of Gerontology Series A: Biological
Sciences and Medical Sciences, 54(8):B352–B357.
ISO 10993-5 (2009). Biological evaluation of medical de-
vices Part 5: Tests for in vitro cytotoxicity.
Klein-Nulend, J., Bakker, A. D., Bacabac, R. G., Vatsa,
A., and Weinbaum, S. (2013). Mechanosensation and
transduction in osteocytes. Bone, 54:2.
Kumagai, K., Takeuchi, R., Ishikawa, H., Yamaguchi, Y.,
Fujisawa, T., Kuniya, T., Takagawa, S., Muschler,
G. F., and Saito, T. (2012). Low-intensity pulsed
ultrasound accelerates fracture healing by stimula-
tion of recruitment of both local and circulating os-
teogenic progenitors. Journal of Orthopaedic Re-
search, 30(9):1516–1521.
Kunnel, J., Gilbert, J., and Stern, P. (2002). In vitro mechan-
ical and cellular responses of neonatal mouse bones to
loading using a novel micromechanical-testing device.
Calcified Tissue International, 71(6):499–507.
Lacroix, D. and Prendergast, P. (2002). Three-dimensional
simulation of fracture repair in the human tibia. Com-
puter Methods in Biomechanics & Biomedical Engi-
neering, 5(5):369–376.
Leung, K. S., Shi, H. F., Cheung, W. H., Qin, L., Ng, W. K.,
Tam, K. F., and Tang, N. (2009). Low-magnitude
high-frequency vibration accelerates callus formation,
mineralization, and fracture healing in rats. Journal of
Orthopaedic Research, 27(4):458–465.
Malizos, K. N., Hantes, M. E., Protopappas, V., and
Papachristos, A. (2006). Low-intensity pulsed ul-
trasound for bone healing: an overview. Injury,
37(1):S56–S62.
Mav
ˇ
ci
ˇ
c, B. and Antoli
ˇ
c, V. (2012). Optimal mechanical
environment of the healing bone fracture/osteotomy.
International Orthopaedics, 36(4):689–695.
Minary-Jolandan, M. and Yu, M.-F. (2009). Nanoscale
characterization of isolated individual type i collagen
fibrils: polarization and piezoelectricity. Nanotech-
nology, 20(8):085706.
NHS-Portugal (2012). Hospital morbidity - Perliminary
data. Portugal: National Health Service.
Niezrecki, C., Brei, D., Balakrishnan, S., and Moskalik, A.
(2001). Piezoelectric actuation: state of the art. The
Shock and Vibration Digest, 33:269.
Perren, S. (1979). Physical and biological aspects of frac-
ture healing with special reference to internal fixa-
tion. Clinical Orthopaedics and Related Research,
138:175–196.
Rath Bonivtch, A., Bonewald, L. F., and Nicolella, D. P.
(2007). Tissue strain amplification at the osteocyte la-
cuna: a microstructural finite element analysis. Jour-
nal of biomechanics, 40(10):2199–2206.
Roseiro, L. M., Neto, M., Amaro, A., Leal, R. P., and
Samarra, M. C. (2013). External fixator configura-
tions in tibia fractures: 1d optimization and 3d analy-
sis comparison. Computer Methods and Programs in
Biomedicine, 113:360–370.
Rubin, C. T., Sommerfeldt, D. W., Judex, S., and Qin, Y.-X.
(2001). Inhibition of osteopenia by low magnitude,
high-frequency mechanical stimuli. Drug Discovery
Today, 6(16):848–858.
Saffar, K. P., JamilPour, N., and Rajaai, S. M. (2009).
How does bhe bone shaft geometry affect its bending
properties? American Journal of Applied Sciences,
6(3):463.
Smith, G. K. (1985). Textbook of small animal orthopedics.
Lippincott Williams & Wilkins.
Stevenson, S. (1998). Enhancement of fracture healing with
autogenous and allogeneic bone grafts. Clinical Or-
thopaedics and Related Research, 355:S239–S246.
Tanaka, S. M. (1999). A new mechanical stimulator for cul-
tured bone cells using piezoelectric actuator. Journal
of Biomechanics, 32(4):427–430.
Tarnit¸
˘
a, D., Tarnit¸
˘
a, D., Popa, D., Grecu, D., TarniT¸
˘
A, R.,
Niculescu, D., and Cismaru, F. (2010). Numerical
simulations of human tibia osteosynthesis using mod-
ular plates based on nitinol staples. Romanian Journal
of Morphology and Embryology, 51(1):145.
Wehner, T., Claes, L., and Simon, U. (2009). Internal loads
in the human tibia during gait. Clinical Biomechanics,
24(3):299–302.
Wu, N., Lee, Y.-c., Segina, D., Benjamin, N., Boulanger,
L., Murray, H., and Wilcox, T. (2013). Cost savings
associated with the use of electrical bone growth stim-
ulation to treat diabetic patients in the us with fracture
nonunion. Journal of Diabetes & Metabolism, 4:4.
Zamora-Navas, P., Verdera, A., Lorenzo, R., Ayuso, J.,
and Reina, M. (1995). Electrical stimulation of bone
nonunion with the presence of a gap. Acta Orthopaed-
ica Belgica, 61:169–176.
EnhancedBoneHealingThroughMechanicalStimulationbyImplantedPiezoelectricActuators
17