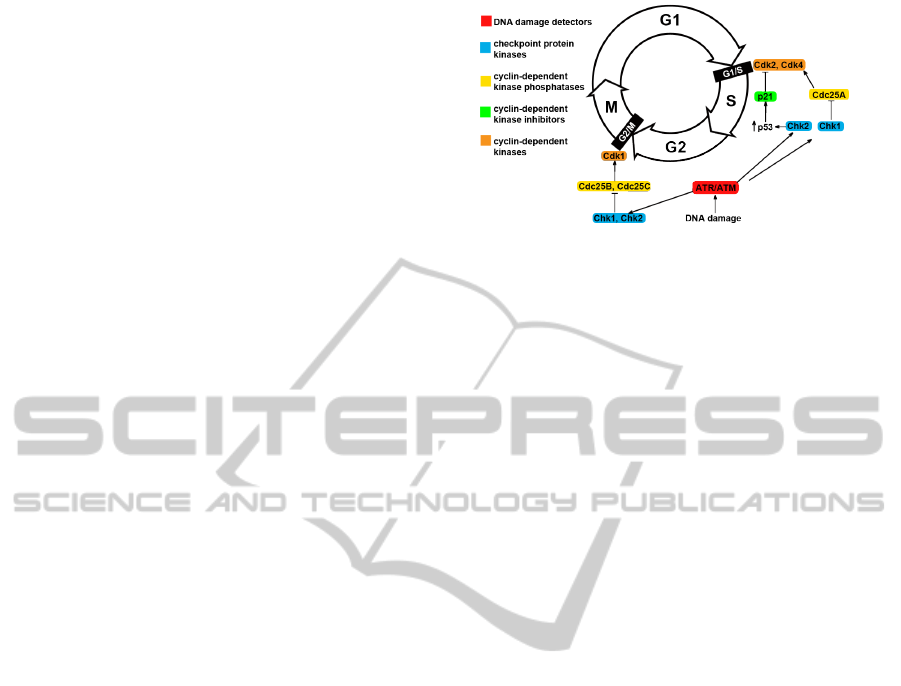
G
1
phase differs even between cells of the same
species.
• S Phase - begins with DNA synthesis, and lasts
for a similar period of time in all cells. The pur-
pose of the processes taking place in the S phase is
to double the amount of DNA present in the cell.
Each chromosome has been replicated. RNA and
protein synthesis in this phase of the cycle is very
slow (with the exception of histone protein syn-
thesis).
• G
2
phase - in this phase synthesis of proteins is
increased again, mainly those responsible for the
formation of the mitotic spindle (tubulin), which
are necessary for the occurrence of a subsequent
process of mitosis.
• G
0
Phase - eukaryotic cells (especially those fully
differentiated) may move from the G1 phase to the
G0 phase, where they do not undergo divisions
and can remain for a long period of time. The
aging of cells in response to damage is a process
that prevents (without causing apoptosis) transfer
of incorrect genetic material to progeny cells.
4.2 Cell Cycle Checkpoints
Cell cycle checkpoints (fig. 5) are mechanisms which
verify correctness of the DNA. If genetic material is
damaged or not all cellular processes specific for each
phase have been completed, cell cycle progression is
stopped until all will be finished and repaired. If the
damage is too big that could have been repaired, cell
is directed to apoptosis (Cooper, 2000; Shapiro and
Harper, 1999). The main checkpoints in the eukary-
otic cells are:
• G
1
/S checkpoint - at the end of G
1
phase (before
synthesis phase) decision whether cell should di-
vide, delay division or enter a G
0
phase is taken.
• G
2
/M checkpoint - occurs at the end of G
2
phase
(before mitosis) and checks if cell is ready to mi-
tosis (whether mitotic apparatus is fully formed
and whether DNA lesions occur).
4.3 Cyclins, Cyclin-Dependent Kinases,
Cyclin-Dependent Kinase
Phosphatases and
Cyclin-Dependent Kinase Inhibitors
To move to next phases of cell cycle is needed co-
operation of two types of molecules: cyclin and
cyclin-dependent kinases. Cyclins and the cyclin-
dependent kinases (CDKs) form together the active
Figure 5: Cell cycle checkpoints and DNA damage detec-
tion.
heterodimer, where cyclins represent a regulatory unit
and are synthesized in specific phases of the cell cy-
cle in response to various molecular signals. CDKs
play a catalytic function and their expression is inde-
pendent of phase of cell cycle. CDKs upon binding to
cyclins are activated and performs the target protein
phosphorylation reactions, which thus become acti-
vated or inactivated, what coordinate entry into the
next phase of the cell cycle. CDKs are often activated
by cyclin-dependent kinase phosphatases (for exam-
ple Cdc25) - tyrosine phosphatases, which acts by
removing the blocking the CDKs activity phosphate
residues (Orlando et al., 2008). Regulation of CDKs
activity might be performed by CDKs inihibitors (like
p21 encoded by a gene CDKN1) which nhibits the
CDK-cyclin complexes activity. p21 binds to cyclin
E/Cdk2 and cyclin D/Cdk4 complexes and inhibiting
their activity acts as a regulator of the cell cycle in the
G1 phase. p21 gene expression is tightly controlled
by the p53 protein (Gartel and Radhakrishnan, 2005).
4.4 Role of Chk1 and Chk2 in
Checkpoint Mechanism
Between G1 and S phase DNA damage results in the
activation of ATM and ATR and following Chk2 and
Chk1 phosphorylation and next phosphorylation of
p53 and Mdm2, which results in activation and sta-
bilization of p53. Active tetramers act as a transcrip-
tion factor of (among others) p21 protein, which is a
potent inhibitor of cyclin-dependent kinases and pre-
vents cells before the entry to the S phase. In addition,
Chk1 phosphorylates and inactivates Cdc25A phos-
phatase that is essential in CDKs activation.
Blocking of the G2-phase is performed with the
signal transducers ATM or ATR (depending on the
type of damage). They activate Chk1 and Chk2 ki-
nases which phosphorylate Cdc25 phosphatase, caus-
ing its inactivation. This avoids the activation of Cdk1
kinase (encoded by the gene CDC2) necessary to ini-
tiate mitosis (Shapiro and Harper, 1999).
DNADamageDetectionanditsImpactontheCellCycle
71