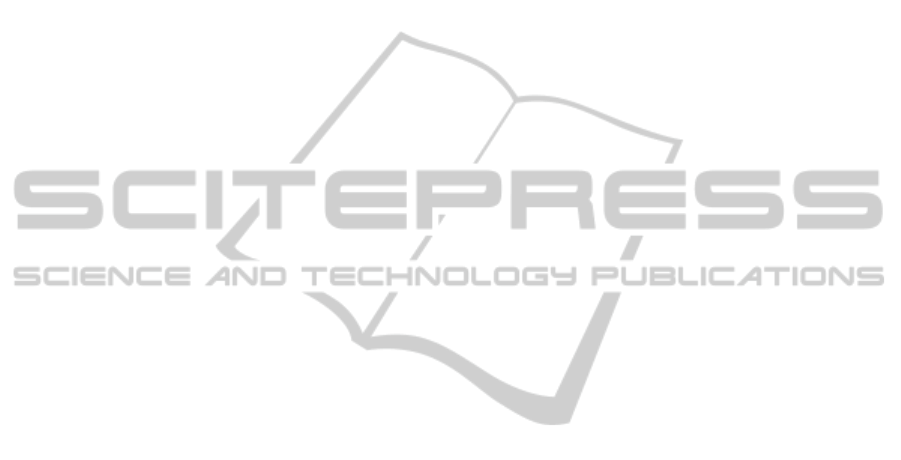
Arrays: A Novel Type of Regenerative Peripheral
Nerve Interface’, IEEE Trans. on Neural Systems and
Rehab. Eng., vol. 17, no.5, pp. 464-460.
Lago N, Ceballos D, Rodríguez FJ, Stieglitz T, and
Navarro X 2005, ‘Long term assessment of axonal
regeneration through polyimide regenerative
electrodes to interface the peripheral nerve’,
Biomaterials, 2005, vol. 26, no.14, pp. 2021-2031.
Lawrence SM, Larsen JO, Horch KW, Riso R, and
Sinkjear T, 2002, ‘Long-Term biocompatibility of
implanted polymer-based intra-fascicular electrodes,’
J. Biomed. Mat. Res., 2002, vol. 63, pp. 501-506.
Lawrence SM, Dhillon GS, Jensen W, Yoshida K and
Horch KW 2004, ‘Acute peripheral nerve recording
characteristics of polymer-based longitudinal
intrafascicular electrodes’, IEEE Trans. Neural. Syst.
Rehabil. Eng., vol. 12, no. 3, pp. 345-348.
Lefurge T, Goodall E, Horch K, Stensaas L and
Schoenberg A 1991, ‘Chronically implanted
intrafascicular recording electrodes’, Annals of
Biomedical Engineering, vol. 19, no. 2, pp. 197-207.
Loeb GE, Marks WB and Beatty PG 1977, ‘Analysis and
microelectronic design of tubular electrode arrays
intended for chronic, multiple single-unit recording
from captured nerve fibres’, Med. Biol. Eng. Comput.,
1977, vol. 15, pp. 195-201.
Loeb GE and Peck RA 1996, ‘Cuff electrodes for chronic
stimulation and recording of peripheral nerve activity’,
J Neurosci Methods, vol. 64, no. 1, pp. 95-103.
Mannard A, Stein RB and Charles D 1974, ‘Regeneration
electrode units: implants for recording from single
peripheral nerve fibers in freely moving animals’,
Science, vol. 183, no. 124, pp. 547-549.
McNaughton TG and Horch KW 1996 ‘Metallized
polymer fibers as leadwires and intrafascicular
microelectrodes’, J. Neurosci. Methods, vol. 70, no.1,
pp. 103-110.
Milkman, R 1998, 'The new American workplace:high
road or low road?' in Workplaces of the future, eds P
Thompson and C Warhurst, Macmillan Press, London,
pp. 22-34.
Naples GG, Mortimer JT, Scheiner A, and Sweeney JD
1988, ‘A spiral nerve cuff electrode for peripheral
nerve stimulation’, IEEE Trans. Biomed. Eng., vol. 35,
pp. 905-916.
Ochoa J and Torebjörk E 1983, ‘Sensations evoked by
intraneural micro-stimulation of single mechano-
receptor units innervating the human hand’, J. Physiol.
vol. 342, pp. 633-654.
Ochoa J and Torebjörk E 1989, ‘Sensations evoked by
intraneural micro-stimulation of C nociceptor fibres in
human skin nerves,’ J Physiol. vol. 415, pp. 583-599.
Riso RR and Slot PJ 1996, ‘Characterization of the ENG
activity from a digital nerve for feedback control in
grasp neuroprostheses’, in Neuroprosthetics: from
Basic Research to Clinical Applications. eds A
Pedotti, M Ferrarin, J Quintern, R Riener, Springer,
Berlin, pp. 345-357.
Riso RR, 1999, ‘Strategies for providing upper extremity
amputees with tactile and hand position feedback-
moving closer to the bionic arm’, Technology in
Health Care, vol. 7, no. 6, pp. 401-409.
Schiefer MA, Freeberg M, Pinault GJ, Anderson J, Hoyen
H, Tyler DJ and Triolo RJ 2013,‘Selective activation
of the human tibial and common peroneal nerves with
a flat interface nerve electrode’, J Neural Eng., vol.
10, no.5, 056006. PubMed PMID: 23918148; PubMed
Central PMCID: PMC3809099.
Schuettler M and Stieglitz T 2000, ‘18polar hybrid cuff
electrodes for stimulation of peripheral nerves’, in:
Proceedings of the 5th Annual Conference of the
International Functional Electrical Stimulation
Society, Alborg, Denmark, pp. 265-268.
Sinkjær T, Haugland M, Struijk J, and Riso RR 1999,
‘Long-term cuff electrode recordings from peripheral
nerves in animals and humans’, in Modern Techniques
in Neuroscience Research. eds U Windhorst and H
Johansson, Springer, Berlin, pp. 787-802.
Stein RB, Charles D, Davis L, Jhamandas J, Mannard A,
and Nichols TR 1975 ‘Principles underlying new
methods for chronic neural recording.’ Can J Neurol
Sci, vol. 2, pp. 235-244.
Stoyanov II, van Wezel RJ and Rutten WL 2013, ‘In vivo
testing of a 3D bifurcating microchannel scaffold
inducing separation of regenerating axon bundles in
peripheral nerves’, J Neural Eng., vol. 10, no. 6,
066018 (13pp)
Tan D, Schiefer M, Keith MW, Anderson R and Tyler DJ
2013, ‘Stability and selectivity of a chronic, multi-
contact cuff electrode for sensory stimulation in a
human amputee’, in Proceedings of the 6
th
International Conference on Neural Engineering
(NER IEEE/EMBS), Nov 6-8, San Diego, CA, pp. 859-
862.
Wallman L, Zhang Y, Laurell T and Danielsen N 2001,
‘The geometric design of micromachined silicon sieve
electrodes influences functional nerve regeneration’,
Biomaterials, 2001. vol. 22, no. 10, pp. 1187-1193.
Walter JS, Griffith P, Sweeney J, Scarpine V, Bidnar M,
McLane J and Robinson C 1997, ‘Multielectrode
nerve cuff stimulation of the median nerve produces
selective movements in a raccoon animal model’, J
Spinal Cord Med., vol. 20, no. 2, pp.233-243.
Wark HA, Sharma R, Mathews KS, Fernandez E, Yoo J,
Christensen B, Tresco P, Rieth L, Solzbacher F,
Normann RA and Tathireddy P, 2013, ‘A new high-
density (25electrodes/mm²) penetrating microelectrode
array for recording and stimulating sub-millimeter
neuroanatomical structures’, J Neural Eng.,vol. 10, no.
4, pp.
NEUROTECHNIX2014-InternationalCongressonNeurotechnology,ElectronicsandInformatics
12