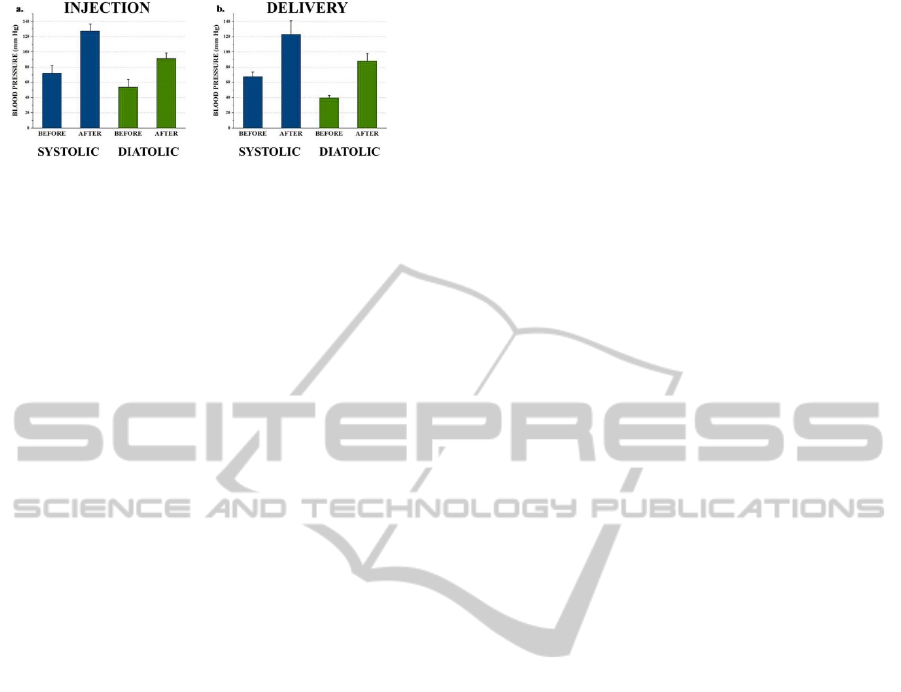
Figure 8: Blood pressure measurement data: a. Drug
delivery by syringe injection. b. Drug delivery by devices.
Both SBP and DBP were measured for every mouse.
5 CONCLUSIONS
Implantable microfluidic drug delivery devices have
been shown to have great potentials in futuristic
cancer treatment. Their controllability and ability to
leverage on the strengths of anti-cancer drugs has
made them an attractive option for overcoming the
present challenges faced in medicine. Demonstrated
with the in vitro pancreatic cancer model, we
concluded that through the programing of these
devices, chemotherapy could potentially be
individualized for every individual to gain better
treatment effects. The biocompatibility and drug
delivery performance of the device were
demonstrated with Kunming mice model in vivo.
Future researches on the microfluidic drug delivery
device will be focused on treatment effects of
different cancer tumor models on small animals, and
further clinical trials.
REFERENCES
Ambati, J., Gragoudas, E. S., Miller, J. W., You, T. T.,
Miyamoto, K., Delori, F. C. & Adamis, A. P. 2000.
Transscleral Delivery Of Bioactive Protein To The
Choroid And Retina. Investigative Ophthalmology &
Visual Science, 41, 1186-1191.
Chen, J., Chu, M., Koulajian, K., Wu, X. Y., Giacca, A. &
Sun, Y. 2009. A Monolithic Polymeric Microdevice
For Ph-Responsive Drug Delivery. Biomedical
Microdevices, 11, 1251-1257.
Chung, A. J., Huh, Y. S. & Erickson, D. 2009. A Robust,
Electrochemically Driven Microwell Drug Delivery
System For Controlled Vasopressin Release.
Biomedical Microdevices, 11, 861-867.
Elman, N. M., Ho Duc, H. L. & Cima, M. J. 2009. An
Implantable Mems Drug Delivery Device For Rapid
Delivery In Ambulatory Emergency Care. Biomedical
Microdevices, 11, 625-631.
Elman, N. M. & Upadhyay, U. M. 2010. Medical
Applications Of Implantable Drug Delivery
Microdevices Based On Mems (Micro-Electro-
Mechanical-Systems). Current Pharmaceutical
Biotechnology, 11, 398-403.
Farra, R., Sheppard, N. F., Mccabe, L., Neer, R. M.,
Anderson, J. M., Santini, J. T., Cima, M. J. & Langer,
R. 2012. First-In-Human Testing Of A Wirelessly
Controlled Drug Delivery Microchip. Science
Translational Medicine, 4, 122ra21.
Gensler, H., Sheybani, R., Li, P.-Y., Lo, R., Zhu, S., Yong,
K.-T., Roy, I., Prasad, P. N., Masood, R. & Sinha, U.
K. Implantable Mems Drug Delivery Device For
Cancer Radiation Reduction. Micro Electro
Mechanical Systems (Mems), 2010 Ieee 23rd
International Conference On, 2010a. Ieee, 23-26.
Gensler, H., Sheybani, R., Li, P. Y., Lo Mann, R. & Meng,
E. 2012. An Implantable Mems Micropump System
For Drug Delivery In Small Animals. Biomedical
Microdevices, 14, 483-496.
Gensler, H., Sheybani, R., Li, P. Y., Lo, R., Zhu, S. T.,
Yong, K. T., Roy, I., Prasad, P. N., Masood, R., Sinha,
U. K., Meng, E. & Ieee 2010b. Implantable Mems
Drug Delivery Device For Cancer Radiation
Reduction. Mems 2010: 23rd Ieee International
Conference On Micro Electro Mechanical Systems,
Technical Digest.
Gottesman, M. M. 2002. Mechanisms Of Cancer Drug
Resistance. Annual Review Of Medicine, 53, 615-627.
Hang Tng, D. J., Song, P., Hu, R., Yang, C. & Yong, K.-T.
2014. High Reliability Nanosandwiched Pt/Ti
Multilayer Electrode Actuators For On-Chip
Biomedical Applications. Analyst, 139, 407-415.
Lavan, D. A., Mcguire, T. & Langer, R. 2003. Small-Scale
Systems For In Vivo Drug Delivery. Nature
Biotechnology, 21, 1184-1191.
Li, P. Y., Sheybani, R., Gutierrez, C. A., Kuo, J. T. W. &
Meng, E. 2010. A Parylene Bellows Electrochemical
Actuator. Journal Of Microelectromechanical Systems,
19, 215-228.
Li, P. Y., Sheybani, R., Kuo, J. T. W. & Meng, E. A
Parylene Bellows Electrochemical Actuator For
Intraocular Drug Delivery. Solid-State Sensors,
Actuators And Microsystems Conference, 2009.
Transducers 2009. International, 21-25 June 2009
2009. 1461-1464.
Li, P. Y., Shih, J., Lo, R., Saati, S., Agrawal, R., Humayun,
M. S., Tai, Y. C. & Meng, E. 2008. An
Electrochemical Intraocular Drug Delivery Device.
Sensors And Actuators A-Physical, 143, 41-48.
Lo, R., Li, P.-Y., Saati, S., Agrawal, R., Humayun, M. &
Meng, E. 2009a. A Passive Mems Drug Delivery
Pump For Treatment Of Ocular Diseases. Biomedical
Microdevices, 11, 959-970.
Lo, R., Li, P. Y., Saati, S., Agrawal, R. N., Humayun, M.
S. & Meng, E. 2009b. A Passive Mems Drug Delivery
Pump For Treatment Of Ocular Diseases. Biomedical
Microdevices, 11, 959-970.
Meng, E. & Hoang, T. 2012. Mems-Enabled Implantable
Drug Infusion Pumps For Laboratory Animal
Research, Preclinical, And Clinical Applications.
Advanced Drug Delivery Reviews, 64, 1628-1638.
BIODEVICES2015-InternationalConferenceonBiomedicalElectronicsandDevices
42