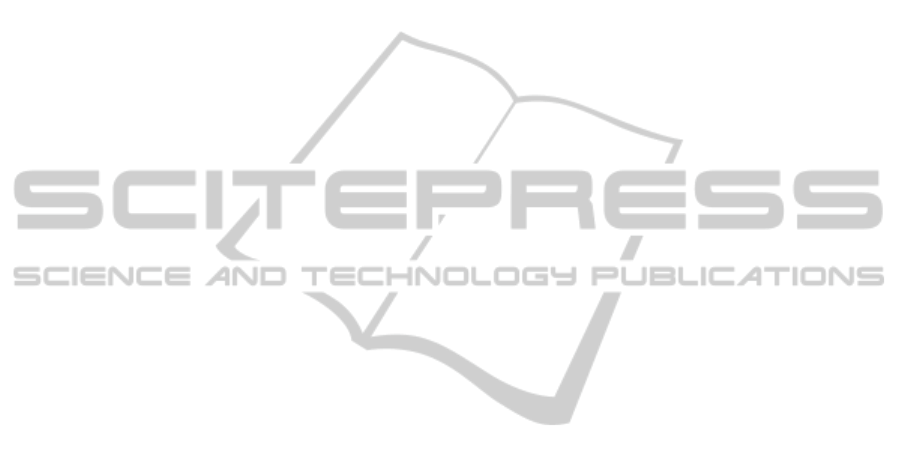
4 CONCLUSIONS
In this work a complete CMOS high frequency
dielectric sensor with hybrid microfluidic integration
was demonstrated. The operating frequency of the
sensor is 12.3 GHz. A sensitivity of 100
MHz/permittivity was measured in the calibration
phase performed with different organic liquids.
Concentration of water in a homogeneous glucose
solution was further measured using the sensor. 250
MHz/10% increase in water content in the glucose
solution was observed. This could be further
interpreted as an approximate increase of 2.5 in the
permittivity of the solution. Concentration of
suspended particles in a solution was further
measured with micro-beads in acetone. Frequency
up-shit of 125 MHz/10 µl increase in bead content in
acetone was measured. All in all a complete CMOS
high frequency sensor with hybrid integrated
microfluidic system was described. The advantages
of using high frequency technique to detect
concentration of particles in a suspension was
investigated and demonstrated in this report.
ACKNOWLEDGEMENTS
The authors would like to thank IHP technology
department for the fabrication of the chip.
REFERENCES
Pires, N.M.M, Dong, T., Hanke, U., Hoivik, N. (2014)
Recent Developments in Optical detection
technologies in Lab-On-a-Chip Devices for
Biosensing Application. Sesnors.14. p. 15458-15479.
Goh, S., and Ram, R.J. (2010) Impedance spectroscopy for
in situ biomass measurements in microbioreactor. 14
th
International conference on Miniaturized Systems and
Life Sciences. Netherlands. p. 1556-1558.
Krommenhoek, E.E., Gardeniers, J., Bomer, J., Van den
Berg, A., Li, X., Ottens, M., van der Wielen, L., van
Dedem, G., Van Leeuwen, M., van Gulik, W.,
Heijnen, J. (2006) Monitoring of yeast cell
concentration using micromachined impedance sensor,
Sensors and Actuators,B. 115.p. 384-389.
Faenza, A., Bocchi, M., Pecorari, N., Franchi, E.,
Guerrieri, R.(2012) Impedance measurement
technique for high sensitivity cell detection in
microstructures with non-uniform conductivity
distribution. Lab Chip 12(11). P.2046-2052.
Rassaei, L., Goluch, E.D., Lemay, S. (2012). Substrate
dependent kinetics in tyrosinase-based biosensing:
Amperometry vs Spectrophotometry. Analytical and
Bioanalytical Chemistry. 493. p.1577-1584.
Grenier, K., Dubuc, D., Chen, T., Artis, F., Chretiennot,
T., Poupot, M., and Fournié, J-J. (2013). Recent
Advances in Microwave-based Dielectric Spectro-
scopy at the Cellular Level for Cancer Investigations
IEEE-TMTT 61(4).p. 2023-2030.
Ferrier, G.A., Romanuik, S.F., Thomson, D.J., Bridges,
G.E., Freeman, M.R. (2009). A microwave interfero-
metric system for simultaneous actuation and detection
of single biological cells. Lab on a Chip. 9. p. 3406-
3412.
Yang, Y., Zhang, H., Zhu, J., Wang, G., Tzeng, T.-R.,
Xuan, X., Huang, K., Wang, P. (2010). Distinguishing
the viability of a single yeast cell with an ultra-
sensitive radio frequency sensor. Lab on a Chip. 10. p.
553-555.
Guha, S., Jamal, F.I., Schmalz, K., Wenger, Ch., Meliani,
Ch. (2013). CMOS lab on a chip device for dielectric
characterization of cell suspensions based on a 6 GHz
Oscillator. European Microwave Conference,
Germany.
Guha, S., Jamal, F.I., Schmalz, K., Wenger, Ch., Meliani,
Ch. (2014) An 8 GHz CMOS Near Field Bio-sensor
Array for Imaging Spatial Permittivity Distribution of
Biomaterials. IEEE MTT-S, Interantional Microwave
Symposium, USA.
T.Lee (1998) The design of CMOS Radio Frequency
Integrated Circuits,Cambridge.
Lisker, M. Trusch, A., Fraschke, M., Kulse, P.,
Borokhovych, Y., Tillack, B., Ostermay, I., Krämer,
T., Schmückle, F.-J., Krüger, O., Krozer, V., Heinrich,
W. (2013). InP-Si BiCMOS Heterointegration Using a
Substrate Transfer Process ECS Transactions.
53(245).
Belrhiti, M.D., Bri, S., Nakheli, A., Haddad, M.,
Mamouni, A. (2012). Dielectric constant determina-
tion of liquid using rectangular waveguide structure
combined with EM simulation. J.Mater.Environ.Sci.
3.p. 575-584.
Kuang, W., Nelson, S.O. (1997). Dielectric relaxation
charcterization of fresh fruits and vegetables from 3 to
20 GHz. J. of Micrwave power and electromagnetic
energy. 32. p. 114-122.
Meriakri, V V., Chigrai, E E., Kim, D., Nikitin, I.P.,
Pangonis, L.I., Parkhomenko, M.P., Won, J.H. (2006).
Dielectric properties of glucose solutions in the
millimetre-wave range and control of glucose content
in blood. IOP, Meas. Sci. Technology. 18.p. 1-6.
Basey-Fisher, T.H., Hanham, S.M., Maier,S.A., Stevens,
M.M., Alford, N.M., Klein, N.( 2011).Microwave
relaxation analysis of Dissolved Proteins:Towards free
solution biosensing. Applied Physics Letters 99.
12GHzCMOSMEMSLab-on-chipSystemforDetectionofConcentrationofSuspendedParticlesinBio-suspensions
57