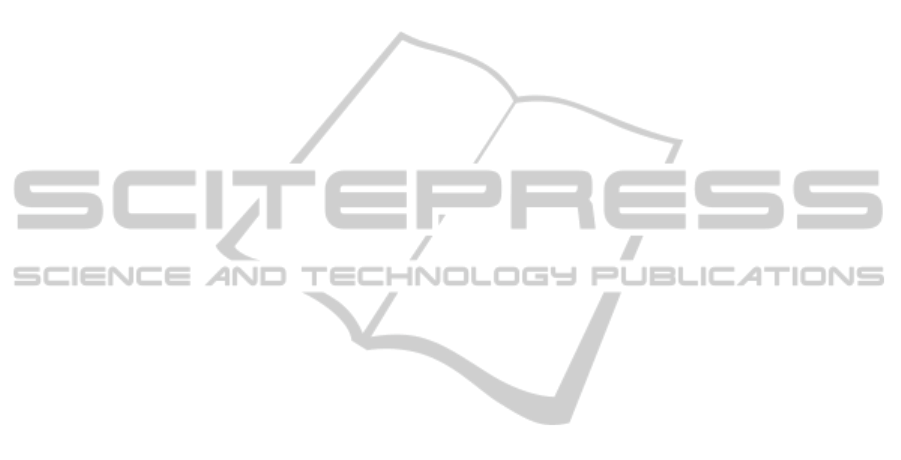
lens increases. Consequently, the focal point is under
control of this parameter and can be adjusted
between 10 and 100 µm, the highest penetration
depth reachable by the excitation beam. The
magnification and the FOV decrease slowly when
the separation distance increases. For example, the
FOV, which is very small at the origin (less than 75
µm) declines to about 73.25 µm at 3 mm
Finally, the coupling efficiency of the
multiphotonic signal emitted by the sample after the
collection by the GRIN lens is not modified
whatever the distance between the fibre and the
GRIN lens. This results from the big inner cladding
diameter of 200 µm with the highest possible NA of
0.3. That optimized parameter is crucial for the
efficient collection of very weak multiphotonic
endogenous signals.
To conclude, these simulations show that if an
axial scanning is needed, moving the separation
distance between the fibre and the GRIN lens is a
way to operate. Nevertheless, one has to expect an
important decrease of the object WD as well as a
modification of the magnification and the FOV.
4 CONCLUSIONS
In the context of the miniaturization of a distal head
of an endomicroscope, GRIN lenses are commonly
used. The aim of this article is to characterize a
commercial GRIN lens. The performances of the
GRIN lens to make images of brain tissues were
tested under a confocal microscope. The presence of
a fluorescent signal emitted by the outlying glue all
around the effective area of the lens was revealed,
and the imaging of glial cells of mouse brain showed
no influence on the resulting fluorescent image of
the sample. Starting from this observation, the
importance of this background fluorescence was
evaluated by spectral analysis and fluorescence
lifetime measurements. Both of them were showing
a non-negligible part of this fluorescence that can be
easily identified by its spectrum and by its lifetime.
The GRIN lens resolutions were then established
to around 1 µm for lateral resolution and 16 µm for
axial resolution, in accordance with the values
usually presented in the literature.
Finally, numerical simulations of an
endomicroscope including this GRIN lens coupled
to a homemade DCF fixed with a PZT scanning
system were led. The limitations of the use of this
PZT were highlighted: the focal plan is necessarily
not plan but curved and the coupling efficiency of
the excitation beam between the DCF and the GRIN
lens is dramatically reduced to less than 40% of
coupling when the fibre angle exceeds 1.4°.
Furthermore, the separation distance between the
DCF and the GRIN lens influenced the position of
the focal point in the object plan. This observation
presents a way to perform an axial scanning system,
which is still a limiting point for obtaining 3D
images. Nevertheless, a modification of the
separation distance induces a reduction of the FOV
and of the magnification. The best simulated FOV
obtained with the GRIN lens is 75 µm square, very
small and far from the ideal situation usually asked
by the surgeons of 1 mm square.
All these limitations have to be carefully thought
for the experimental use of the GRIN lens. It
remains that GRIN lens technology is the only one
allowing a distal lens with a diameter smaller than
0.5 mm, a decisive advantage in nonlinear
endomicroscopy.
ACKNOWLEDGEMENTS
This work has been highly supported by INCA Plan
Cancer with Physicancer program grants “MEMBO”
& “MEVO” and the Institut National de Physique
Nucléaire et de Physique des Particules (IN2P3).
This work was supported by the L’Oreal
Foundation, thanks to the French National Program
“For Woman in Science”, distinguishing Claire
Lefort for her work on endomicroscopy.
REFERENCES
Cosignani, V., Dvornikov, A., Aguilar, J.-S., Stingari, C.,
Edwards, R., Mantulin, W. W., Gratton, E., 2012.
Deep tissue fluorescence imaging and in vivo
biological applications. Journal of Biomedical Optics,
17, 11, 116023.
Ducourthial, G., Lefort, C., Peyrot, D.A., Mansuryan, T.,
Kruglik, S.G., Vever-Bizet, C., Thiberville, L.,
Lacombe, F., Bourg-Heckly, G., Louradour, F., 2013.
Label free multiphoton imaging of human pulmonary
tissues through two-meter-long microstructured fiber
and multicore image-guide. Progress in Biomedical
Optics and Imaging - Proceedings of SPIE, 85750H.
Gu, M., Bao, H., Kang, H., 2014. Fibre-optical
microendoscopy. Journal of Microscopy, 254, 1, pp.
13-18.
Kim, J., Lee, W. M., Kim, P., Choi, M., Jung, K., Kim, S.,
Yun, S. H., 2012. Fabrication and operation of GRIN
probes for in vivo fluorescence cellular imaging of
internal organs in small animals. Nature Protocols, 7,
8, pp 1456-1469.
DesignofanOptimizedDistalOpticforNonLinearEndomicroscopy
11