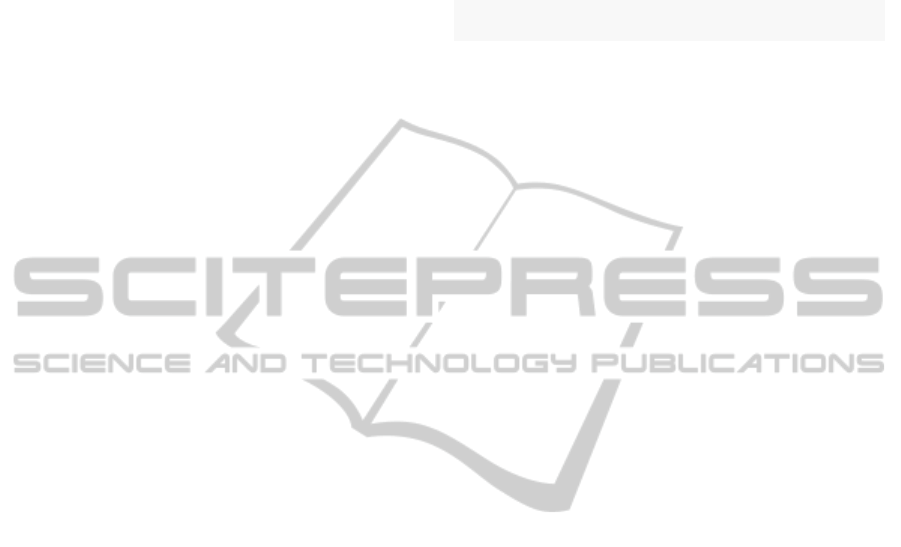
A. Katelnikovas, P. Vitta, P. Pobedinskas, G. Tamulaitis,
A. Zukauskas, J.-E. Jørgensen, and A. Kareiva, 2007.
J. Cryst. Growth 304, 361.
E. Caponetti, M. L. Saladino, F. Serra, and S. Enzo, 2007.
J. Mater. Sci. 42, 4418.
E. Caponetti, S. Enzo, B. Lasio, and M. L. Saladino, 2007
Opt. Mater. 29, 1240.
L. Lipinska, L. Lojko, A. Klos, S. Ganschow, R.
Diduszko, W. Ryba-Romanowski, and A.
Pajaczkowska, 2007. J. All. Comp. 432, 177.
A. Katelnikovas, T. Justel, D. Uhlich, J.-E. Jorgensen, S.
Sakirzanovas, and A. Kareiva. 2008. Chem. Eng.
Comm. 195, 758.
N. N. Khimich, E. N. Poddenezhnyi, A. A. Boiko, A. V.
Zdravkov, V. L. Ugolkov, L. A. Koptelova, E. I.
Grishkova, and A. O. Dobrodei, 2009. Glass Phys.
Chem. 35, 504.
M. Suarez, A. Fernandez, J. L. Menendez, and R.
Torrecillas, 2009. J. Nanomater. Art. # 138490.
H. J. Yang, L. Yuan, G. S. Zhu, A. B. Yu, and H. R. Xu,
2009. Mater. Lett. 63, 2271.
X. X. Ge, Y. H. Sun, C. Liu, and W. K. Qi, 2009. J. Sol-
Gel Sci. Technol. 52, 179.
K. Fujioka, T. Saiki, S. Motokoshi, Y. Fujimoto, H. Fujita,
and M. Nakatsuka, 2009. Ceram. Int. 35, 2393.
H. K. Yang, and J. H. Jeong, 2010. J. Phys. Chem. C 114,
226.
Y. Iida, A. Towata, T. Tsugoshi, and M.Furukawa, 1999.
Vibr. Spectr. 19, 399.
A. Golubovic, S. Nikolic, R. Gajic, S. Duric, and A.
Valcic, 2002. J. Serb. Chem. Soc. 67, 291.
L. Zhang, C. Y. Zhang, D. H. Li, Z. Y. Wei, Z. G. Zhang,
J. E. Hans, and S. Strohmaier, 2008. Chin. Phys. Lett.
25, 3988.
T. Vosegaard, D. Massiot, N. Gautier, and H. J. Jakobsen,
1997. Inorg. Chem. 36, 2446.
B. Ferrand, B. Chambaz, and M. Couchaud, 1999. Opt.
Mater. 11, 101.
R. Gaume, B. Viana, J. Derouet, and D. Vivien, 2003. Opt.
Mater. 22, 107.
I. Muliuoliene, S. Mathur, D. Jasaitis, H. Shen, V.
Sivakov, R. Rapalaviciute, A. Beganskiene, and A.
Kareiva, 2003. Opt. Mater. 22, 241.
S. Chenais, F. Druon, F. Balembois, P. Georges, A.
Brenier, and G. Boulon, 2003. Opt. Mater. 22 99.
E. Garskaite, Z. Moravec, J. Pinkas, S. Mathur, R.
Kazlauskas, and A. Kareiva, 2005. Phil. Magaz. Lett.
85, 557.
S. Mathur, H. Shen, A. Leleckaite, A. Beganskiene, and A.
Kareiva, 2005. Mater. Res. Bull. 40, 439.
S. Sakirzanovas, L. Sun, Ch. Yan, and A. Kareiva, 2008.
Mendeleev Commun. 18, 251.
A. Katelnikovas, and A. Kareiva. 2008. Mater. Lett. 62,
1655.
K. Papagelis, G. Kanellis, T. Zorba, S. Ves, and G. A.
Kourouklis, 2002. J. Phys. Condens. Matter 14, 915.
K. Papagelis, and S. Ves, 2003. J. Phys. Chem. Solids 64,
599.
C. Milanese, V. Buscaglia, F. Maglia, and U. Anselmo-
Tamburini, 2004. Chem. Mater. 16, 1232.
A. Katelnikovas, J. Barkauskas, F. Ivanauskas, A.
Beganskiene, and A. Kareiva, 2007. J. Sol-Gel Sci.
Techn. 41, 193.
A. Kareiva, 2011. Materials Science (Medžiagotyra), 17,
428.
R. Skaudzius, A. Katelnikovas, D. Enseling, A. Kareiva,
and T. Juestel, J. Lumin. 147, 290 (2014).
A. Zabiliute, S. Butkute, A. Zukauskas, P. Vitta, and A.
Kareiva, 2014. Appl. Optics, 53, 907.
J. Livage, M. Henry, C. Sanchez, 1988. Progr. Solid State
Chem. 18, 259.
C. J. Brinker, G. W. Scherrer, 1990. Sol-Gel Science: The
Physics and Chemistry of Sol-Gel Processing,
Academic Press, San Diego.
B. L. Cushing, V. L. Kolesnichenko, and C. J. O‘Connor,
2004. Chem. Rev. 104, 3893.
J. D. Mackenzie, and E. P. Bescher, 2007. Acc. Chem.
Res. 40, 810.
N. Dubnikova, E. Garskaite, J. Pinkas, P. Bezdicka, A.
Beganskiene, A. Kareiva, 2010. J. Sol-Gel Sci. Techn.
55 (2), p.213 – 219.
E. Garskaite, N. Dubnikova, A. Katelnikovas, J. Pinkas,
and A. Kareiva, 2010. Collect. Czech. Chem.
Commun. 72, 321.
Xia Li, Hong Liu, Jiyang Wang, Hongmei Cui, Shunliang
Yang, I.R. Boughton, 2005. J. Phys. Chem. Solids 66
201-205.
Xia Li, Hong Liu, Jiyang Wang, Hongmei Cui, Feng Han,
2004. J. Am. Ceram. Soc., 87 [12] 2288-2290.
Lin Xing, Luming Peng, Min Gu, Guodong Tang, 2004. J.
Alloys Comp. 491 599-604.
Nathalie Pralad, Genevieve Chadeyron, Audrey Potdevin,
Jerome Deschamps, Rachid Mahiou, 2013. J. Eur.
Ceram. Soc. 33 1935-1945.
R. Skaudzius, A. Zalga, and A. Kareiva, 2008. Materials
Science - Medziagotyra 14, 193.
G.Seeta Rama Raju, S. Buddhudu, 2008. Mater. Lett. 62
1259.
Jin Young Park, Hong Chae Jung, G. Seeta Rama Raju,
Byung Kee Moon, Jung Hyun Jeong, Jung Hwan Kim.
2010. J. Lumin. 130 478 – 482.
R. Srinivasan, R. Yogamalar, A. Chandra Bose, 2010.
Meter. Res Bull. 45.
J. Dhanaraj, R. Jagannathan, T.R.N. Kutty, C.-Hsin Lu,
2001. J. Phys. Chem. B 105 11098.
P.Packiyaraj, P. Thangadurai, 2014. J. Lumin 145 997-
1003.
S. K. Singh, Dong Gi Lee, Soung Soo Yi, Kiwan Jang,
Dong-Soo Shi, Jung Hyun Jeong, 2013. J. Appl. Phys.
113, 173504.
Redenka M., Krsmanovič Whiffen, Željka Antic, Adolfo
Speghini, Mikhail G. Brik, Barbora Bartova, Marco
Bettinelli, Miroslav D. Dramicanin, 2014. Opt. Mater.
36 1083-1091.
P.Packiyaraj, P. Thangadurai, 2014. J. Lumin 145 997-
1003.
R. E.Muenchausen, L. G. Jacobsohn, B. L. Bennett, E. A.
McKigney, 2007. J. F. Smith, J. A. Valdez, D. W.
Cooke, J. Lumin, 126 838.
OntheSol-gelPreparationofSelectedLanthanideAluminiumGarnetsDopedwithEuropium
171