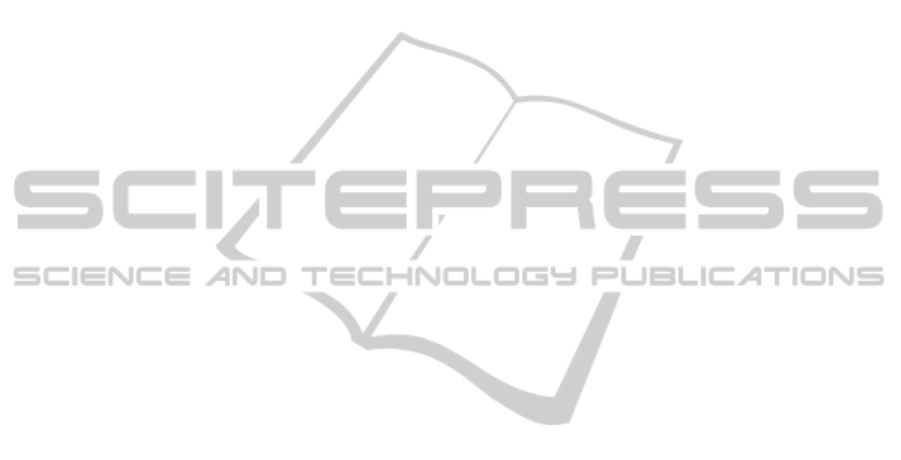
ACKNOWLEDGEMENTS
This work has been highly supported by the LABEX
program of XLIM Institute in the axis “Biophysics
and health”, the Limousin Region and Limoges
University for the transversal thematic “Bio-Electro-
Photonic”. We also thank Fibrecore Inc. for providing
30 meters of the single mode fibre for free.
REFERENCES
Ali, M. A., Moghaddasi, J., Ahmed, S. A., 1990.
Temperature effects in rhodamine b dyes and
improvement in cw dye laser performance, Laser
Chem., 11, pp. 31-38.
Baffou, G., Rigneault, H., Marguet, D., Jullien, L., 2014. A
critique of methods for temperature imaging in single
cells, Nature Methods, 11, 9, pp. 899-901.
Chapman, C. F., Liu, Y., Sonek, G. J., Tromberg, B. J.,
1995. The use of exogenous fluorescent probes for
temperature measurements in single living cell,
Photochemistry and photobiology, 62, 3, pp 416-425.
Chen, Y.Y., Wood, A.W., 2009. Application of a
temperature-dependent fluorescent dye (Rhodamine B)
to the measurement of radiofrequency radiation-
induced temperature changes in biological samples,
Bioelectromagnetics, 30, 7, pp. 583–590.
Donner, J.S., Thompson, S.A., Kreuzer, M.P., Baffou, G.,
Quidant, R., 2012. Mapping Intracellular Temperature
Using Green Fluorescent Protein, Nano Letters, 12, 4,
pp. 2107-2111.
Ferguson, J. and Mau, AWH, 1973. Spontaneous and
stimulated emission from dyes. Spectroscopy of the
neutral molecules of acridine orange, proflavine, and
rhodamine B, Australian Journal of Chemistry 26, 8,
pp. 1617 – 1624.
Gui, L., Ren, C.L., 2008. Temperature measurement in
microfluidic chips using photobleaching of a
fluorescent thin film, Applied Physics Letters, 92, 2.
Hoover, E.E., Squier, J.A., 2013. Advances in multiphoton
microscopy technology, Nature Photonics Review, 7,
pp. 93-101.
Kohler, S., O’Connor, R.P., Thi Dan Thao Vu, Leveque, P.,
Arnaud-Cormos, D., 2013. Experimental
microdosimetry techniques for biological cells exposed
to nanosecond pulsed electric fields using
microfluorimetry, IEEE Transactions on Microwave
Theory and Techniques, 61, 5.
Kubin, R. F., and Fletcher, A. N., December 1982–February
1983. Fluorescence quantum yields of some rhodamine
dyes, Journal of Luminescence, 27, 4, pp. 455–462.
Li, B.-H., Xie, S.-S., Huang, Z., Wilson, B.C., 2009.
Advances in photodynamic therapy dosimetry. Progress
in Biochemistry and Biophysics, 36, 6, pp. 676-683.
Liljemaln, R., Nyberg, T., von Holst, H., 2013. Heating
during neural stimulation, Lasers in Surgery and
Medicine, 45, pp. 469-481.
Löw, P., Kim, B., Takama
,
N., Bergaud,C., 2008. High-
Spatial-Resolution Surface-Temperature Mapping
Using Fluorescent Thermometry, Small, 4, 7, pp. 908-
914.
Martinez Maestro, L., Rodriguez, E.M., Sanz Rodriguez,
F., Iglzsias-de la Cruz, M.C., Juarranz, A., Naccache,
R., Vetrone, F., Jaque, D., Capobianco, J.A., Garcia
Sole, J., 2010. CdSe Quantum Dots for Two-Photon
Fluorescence Thermal Imaging, Nano Letters, 10, 12,
pp. 5109-5115.
Okabe, K., Inada, I., Gota, C., Harada, Y., Funatsu, T.,
Uchiyama, S., 2012. Intracellular temperature
mapping with a fluorescent polymeric thermometer and
fluorescence lifetime imaging microscopy, Nature
Communication, 3, 705, pp. 1-9.
Reungpatthanaphong, P., Dechsupa, S., Meesungnoen, J.,
Loetchutinat, C., Mankhetkorn,S., 2003. Rhodamine B
as a mitochondrial probe for measurement and
monitoring of mitochondrial membrane potential in
drug-sensitive and -resistant cells, Journal of
Biochemical and Biophysical Methods, 57, 1, pp. 1-16.
Richter, C.-P., and Tan, 2014. Photons and neurons,
Heating research, 311, pp. 72-88.
Ross, D., Gaitan, M. and Locascio, L.E., 2001.
Temperature measurement inmicrofluidic systems
using a temperature-dependent fluorescent dye,
Analytical Chemistry, 73, pp. 4117–4123.
Sakakibara, J., and Adrian, R.J., 1999. Whole field
measurement of temperature in water using two-color
laser induced fluorescence, Exper.Fluids, 26, pp. 7–15.
Shah, J. J., Gaitan, M., Geist, J., 2009. Generalized
temperature measurement equations for rhodamine b
dye solution and its application to microfluidics,
Analytical Chemistry, 81, 19, pp 8260–8263.
Shah, J. J., Sundaresan, S. G., Geist, J., Reyes, D. R., Booth,
J. C. Mulpuri, Rao, V., Gaitan,M., 2007. Microwave
dielectric heating of fluids in an integrated microfluidic
device, Journal of Micromechanics and
Microengineering, 17, 11.
Shang, L., Stockmar, F., Azadfar, N., Nienhaus, G.U.,
2013. Intracellular Thermometry by Using Fluorescent
Gold Nanoclusters, Angewandte Chemie International
Edition, 52, 42, pp. 11154–11157.
Vetrone, F., Naccache, R., Zamarron, A., Juarranz de la
Fuente, A., Sanz-Rodriguez, F., Marinez, L.,
Rodriguez, E.M., Jaques, D., Garcia Sole, J.,
Capobianco, J.A., 2010. Temperature sensing using
fluorescence nanothermometers, ACS Nano, 4, 6, pp.
3254-3258.
Welch, A.J., 1984. The thermal response of laser irradiated
tissues, IEEE Journal of Quantum Electronics, 20, 12
pp. 1471-1481.
Yang, L., Peng, H.-S., Ding, H., You, F.-T., Hou, L.-L.,
Teng, F., 2013. Luminescent Ru(bpy)3 2+-doped silica
nanoparticles for imaging of intracellular temperature,
Microchimica Acta, 181, 7-8, pp. 743-749.
Zohar, O., Ikeda, M., Shinagawa, H., Inoue, H., Nakamura,
H., Elbaum, D., Alkon, D.L., Yoshioka, T., 1998.
Thermal imaging of receptor-activated heat production
in single cells, Biophysical Journal, 74, 1, pp. 82-89.
PHOTOPTICS2015-InternationalConferenceonPhotonics,OpticsandLaserTechnology
52