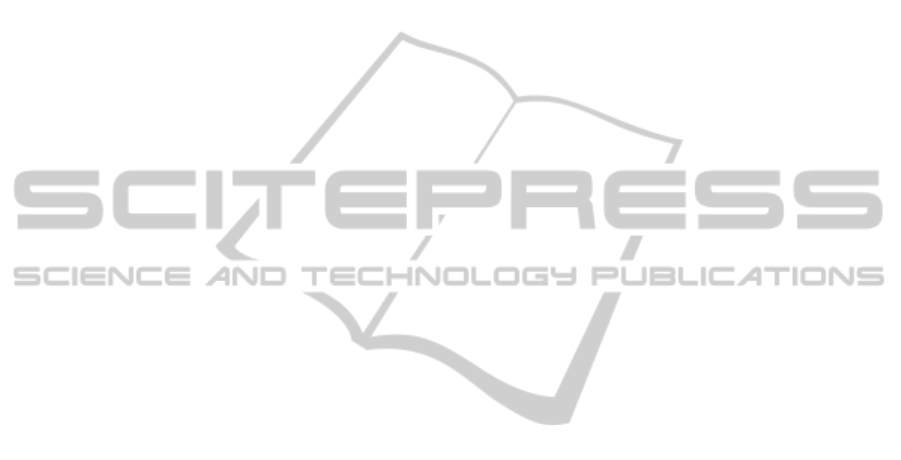
obtained also since a QPM array structure is used.
The proposed device has a compact structure and can
be operated with a low millimetre-wave signal loss
and no external electrical power supply.
The proposed device is promising for broadband
wireless communication such as for Multi-Input
Multi-Output (MIMO) and Space-Division-
Multiplexing-Access (SDMA) (Wijayanto, 2013). It
can be used also for precise measurement/ sensing
applications such as high-frequency Electromagnetic
Compatibility (EMC) chamber and Radio Detecting
and Ranging (RADAR).
ACKNOWLEDGEMENTS
The authors would like thank to Dr. T. Umezawa
from National Institute of Information and
Communication Technology (NICT) Japan and Dr.
H. Shiomi from Osaka University Japan for their
constructive comments and suggestions during
discussion. Thanks to Dr. Y. Ogawa from NICT
Japan for his helpful supports during device
fabrication.
Y. N. Wijayanto, A. Kanno, S. Nakajima, and T.
Kawanishi would like thank to the Ministry of
Internal Affairs and Communications, Japan, for the
financial support partly thru the project entitled
“Research and Development of high-precision
imaging technology using 90 GHz band linear cells”
funded by the “Research and Development to Expand
Radio Frequency Resources.”
REFERENCES
Abichar, Z., Peng, Y., and Chang, J., “WiMAX: The
Emergence of Wireless Broadband,” IEEE Computer
Society, Vols. July-August, pp. 44-48, 2006.
Akyildiz, I. F., Gutierrez-Estevez, D.M., Reyes, E.C., “The
evolution to 4G cellular systems: LTE-Advanced,”
Physical Communication, vol. 3, pp. 217-244, 2010.
Bridges, W. B., Sheehy, F.T., and Schaffner, J.H., “Wave-
Coupled LiNbO
3
Modulator for Microwave and
Millimeter-Wave Modulation,” IEEE Photonics
Technology Letters, vol.3, no.2, pp.133-135, February
1991.
Gupta, V. R. and Gupta, N., “Characteristics of a Compact
Microstrip Antenna,” Microwave and Optical
Technology Letters, vol.40, no.2, pp.158-160, January
2004.
Hu, H., Ricken, R., Sohler, W., "Low-loss ridge
waveguides on lithium niobate fabricated by local
diffusion doping with titanium," Applied Physics B,
vol.98, pp.677–679, 2010.
Iezekiel, S., Microwave photonics: Devices and
Applications, John Wiley & Sons Ltd, Chichester, UK,
2009.
Lefort, G., and Razban, T., “Microstrip Antennas Printed
on Lithium Niobate Substrate,” Electronics Letters,
vol.33 no.9, pp.726-727, April 1997.
Lu, X., Wang, P., Niyato, D., and Hossain, E., “Dynamic
Spectrum Access in Cognitive Radio Networks with RF
Energy Harvesting,” IEEE Wireless Communications,
pp. 102-110, Jun. 2014.
Mendeiros, H. P., Maciel, M. C., Sauza, R. D., and Pellenz,
M.E., “Lightweight Data Compression in Wireless
Sensor Networks Using Human Coding,” International
Journal of Distributed Sensor Networks, vol. 2014, ID
672921, Jan. 2014.
Murata, H., Miyanaka, R., and Okamura, Y., “Wireless
Space-Division-Multiplexed Signal Discrimination
Device Using Electro-Optic Modulator with Antenna-
Coupled Electrodes and Polarization-Reversed
Sructures,” International Journal of Microwave and
Wireless Technologies, vol.4, pp.399-405, April 2012.
Pi, Z. and Khan, F., “An introduction to millieter-wave
mobile broadband systems,” IEEE Communications
Magazine, vol. June 2011, pp. 101-107, 2011.
Recsi. ITU-R P.676-5, “Attenuation of atmospheric gases,”
2001.
Rodriguez-Berral, R., Mesa, F., and Jackson, D. R., “Gap
Discontinuity in Microstrip Lines: An Accurate Semi
analytical Formulation,” IEEE Transactions on
Microwave Theory and Techniques, vol.59, no.6, pp,
1441-1453, June 2011.
Shi, J., Huang, C., and Pan, C., “Millimeter-wave photonic
wireless links for very high data rate communication,”
NPG Asia Materials, vol.3, pp.41, April 2011.
Seeds, A. J., “Microwave Photonics,” IEEE Transactions
on Microwave Theory and Techniques, vol.50, no.3,
pp.877-887, March 2002.
Shinada, S., Kawanishi, T., and Izutsu, M., “A Resonant
Type LiNbO
3
Optical Modulator Array with Micro-
Strip Antennas,” IEICE Transactions on Electronics,
vol.E90-C, no.5, pp.1090-1095, May 2007.
Sheehy, F. T., Bridges, W. B., and Schaffner, J. H., “60
GHz and 94 GHz Antenna-Coupled LiNbO
3
Electrooptic Modulators,” IEEE Photonics Technology
Letters, vol.5, no.3, pp.307-310, March 1993.
Uddin, M. A., Chan, H. P., Tsun, T. O., and Chan, Y. C.,
"Uneven Curing Induced Interfacial Delamination of
UV Adhesive-Bonded Fiber Array in V-Groove for
Photonic Packaging," Journal of Lightwave
Technology, vol.24, no.3, pp.1342-1349, March 2006.
Watanabe, I., Nakata, T., Tsuji, M., Makita, K, Torikai, T.,
and Taguchi, K., “High-Speed, High-Reliability Planar-
Structure Superlattice Avalanche Photodiodes for 10-
Gb/s Optical Receivers,” Journal of Lightwave
Technology, vol.18, no.12, pp. 2200- 2207, December
2000.
Wijayanto, Y. N., Murata, H., and Okamura, Y., “Novel
Electro-Optic Microwave-Lightwave Converters
Utilizing a Patch-antenna Embedded with a Narrow
PHOTOPTICS2015-InternationalConferenceonPhotonics,OpticsandLaserTechnology
12