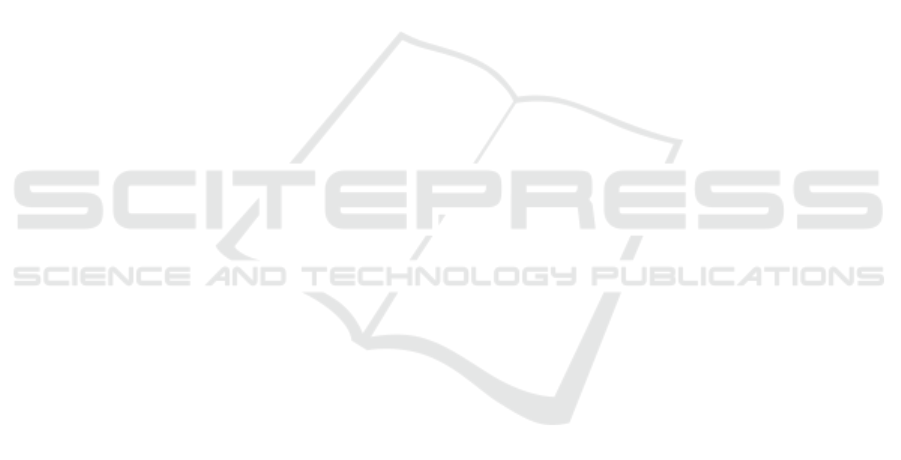
Periodic and Metallic Nano-structures Patterned by Contact Transfer
Lithography with Application on Localized Surface Plasmon
Resonance
Hao-Yuan Chung, Chun-Ying Wu and Yung-Chun Lee
Department of Mechanical Engineering, National Cheng-Kung University, Tainan, Taiwan
Keywords: Localized Surface Plasma Resonance, Metallic Nano-structures, Contact Transfer Lithography.
Abstract: In this study, we demonstrate a rapidly, low cost, and mass production process to fabricate arrayed metallic
nanoparticles on a variety of substrates based on contact transfer and metal mask embedded lithography
(CMEL). A hexagonal arrayed metallic nanoparticles deployed on ITO/glass substrate with sub-micron
periodicity is achieved. It is observed in optical transmittance measurements that noble metallic arrayed
nanoparticles deployed on ITO/glass substrate result in a spectrally narrowband of extinction in visible
range, and is in good agreement with the simulated results using finite-element method (FEM). It is found
that the narrowband extinction spectrum is associated with electromagnetic field coupling between the
arrayed metallic nanostructures and the ITO layer. This electromagnetic field coupling induces significant
plasmon resonance in the ITO layer underneath the arrayed metallic nanostructures. Based on this observed
phenomenon and our innovative large-area nano-fabrication processes, optoelectronic devices with arrayed
metallic nanostructures can be easily designed and developed.
1 INTRODUCTION
Arrayed metallic nanoparticles have gained lots of
attentions in both scientific researches as well as
engineering application during last few decades.
Metallic nanostructures exhibit a rich variety of
intriguing optical properties due to the interaction of
the electromagnetic field with the free electrons of
the metal. Such an excitation can occur at a metal-
dielectric interface and is called surface-plasmon
polariton or at a metallic nanoparticle, and in this
case it is termed as particle-plasmon polariton
(Linden, Kuhl, and Giessen, 2001; Hutter and
Fendler, 2004; Yannopapas and Stefanou, 2004)
There are several ways to achieve metallic
nanoparticles, such as laser ablation method,
chemical reduction method and pyrolysis method
(Mafune et al., 2001; Pillai et al., 2007). These
methods can produce nanoparticles over large area
but have limitations to efficiently deploy
nanoparticles in specified arrangements. Electron
beam lithography is an excellent method to fabricate
arrayed metallic nanoparticles. The size of
nanoparticle can be well controlled to about several
tens of nanometers and arranged into square or
triangular lattice (Linden, Kuhl, and Giessen, 2001).
However, the costs of equipment and time-
consuming issues limit the capability to mass
produce large-area devices.
In this study, we demonstrate a rapidly, low cost,
and mass productive process to deploy arrayed
metallic nanoparticles on a variety of substrates
based on contact-transfer and mask-embedded
lithography (CMEL). A hexagonal arrayed metallic
nanoparticles deployed on an ITO/glass substrate
with sub-micron periodicity is obtained. Moreover,
the optical transmittance spectrum of the sample is
measured via spectrophotometer experimentally and
a numerical simulation using finite-element method
(FEM) is carried out to identify the mechanisms of
observed resonance characteristics.
2 EXPERIMENTAL DETAILS
AND RESULTS
This section describes the experimental details to
fabricate arrayed metallic nanoparticles on a variety
of substrates. A nanoimprinting process presented in
our previous study (Lee and Chiu, 2008), CMEL, is
applied to define and pattern arrayed metallic
structures which have feature sizes in sub-micron or
nanometer scales. First of all, a hexagonal arrayed
20
Chung H., Wu C. and Lee Y..
Periodic and Metallic Nano-structures Patterned by Contact Transfer Lithography with Application on Localized Surface Plasmon Resonance.
DOI: 10.5220/0005333500200025
In Proceedings of the 3rd International Conference on Photonics, Optics and Laser Technology (PHOTOPTICS-2015), pages 20-25
ISBN: 978-989-758-092-5
Copyright
c
2015 SCITEPRESS (Science and Technology Publications, Lda.)