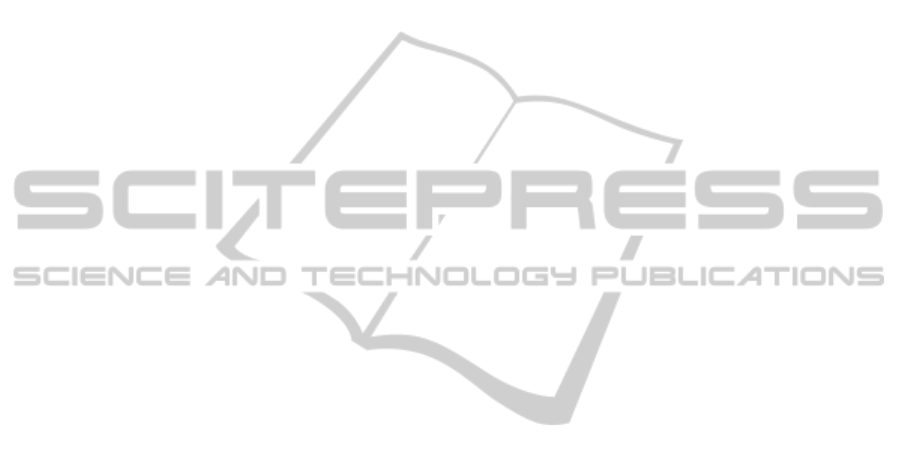
Gautier, S. et al., 2007. GaN materials growth by MOVPE
in a new-design reactor using DMHy and NH3.
Journal of Crystal Growth, 298, pp.428–432.
Gil, B., 1998. Group III nitride semiconductor compounds,
Clarendon Press.
Han, J. et al., 1998. AlGaN/GaN quantum well ultraviolet
light emitting diodes. Applied Physics Letters, 73(12),
p.1688.
Higuchi, Y. et al., 2008. Room-temperature CW lasing of
a GaN-based vertical-cavity surface-emitting laser by
current injection. Applied Physics Express, 1,
p.121102 1–3.
Hirayama, H. et al., 2007. 231–261 nm AlGaN deep-
ultraviolet light-emitting diodes fabricated on AlN
multilayer buffers grown by ammonia pulse-flow
method on sapphire. Applied Physics Letters, 91(7),
p.71901.
Hirayama, H. et al., 2014. Recent progress and future
prospects of AlGaN-based high-efficiency deep-
ultraviolet light-emitting diodes. Japanese Journal of
Applied Physics, 53(10), p.100209.
Ippommatsu, M. et al., 2013. Development of AlGaN
DUV-LED. In 10th Conference on Lasers and
Electro-Optics Pacific Rim (CLEO-PR).
Ivanov, S.V. et al., 2014. Plasma-assisted molecular beam
epitaxy of Al(Ga)N layers and quantum well
structures for optically pumped mid-UV lasers on c-
Al
2
O
3
. Semiconductor Science and Technology, 29(8),
p.084008.
Johnson, N.M. et al., 2012. In paper presented at the 9th
International Symposium on Semiconductor Light
Emitting Devices, Berlin.
Kasahara, D. et al., 2011. Demonstration of blue and
green GaN-based vertical-cavity surface-emitting
lasers by current injection at room temperature.
Applied Physics Express, 4(7), p.072103 1–3.
Kim, J. et al., 2014. Origins of unintentional incorporation
of gallium in InAlN layers during epitaxial growth,
part II: Effects of underlying layers and growth
chamber conditions. Journal of Crystal Growth, 388,
p.143-149.
Kolbe, T. et al., 2010. Optical polarization characteristics
of ultraviolet (In)(Al)GaN multiple quantum well
light emitting diodes. Applied Physics Letters, 97(17),
p.171105.
Krestnikov, I.L. & Ledentsov, N.N., 1999. Photopumped
InGaN / GaN / AlGaN Vertical Cavity Surface
Emitting Laser Operating at Room Temperature.
phys. stat. sol., 511, pp.511–516.
Leroux, M. et al., 2004. About some optical properties of
Al
x
Ga
1−x
N /GaN quantum wells grown by molecular
beam epitaxy. Superlattices and Microstructures, 36,
pp.659–674.
Leroux, M. et al., 2002. Optical characterization of
Al
x
Ga
1-x
N alloys ( x<0.7 ) grown on sapphire or
silicon. Physica Status Solidi (b), 234(3), pp.887–891.
Li, X., Sundaram, S., Disseix, P., et al., 2015. AlGaN-
based MQWs grown on a thick relaxed AlGaN buffer
on AlN templates emitting at 285 nm. Optical
Materials Express, 5(2), pp.380-392.
Li, X., Sundaram, S., El Gmili, Y., et al., 2014. BAlN thin
layers for deep UV applications. In E-MRS Spring
Meeting.
Li, X., Sundaram, S., El Gmili, Y., et al., 2014. MOVPE
grown periodic AlN/BAlN heterostructure with high
boron content. Journal of Crystal Growth, pp.3–6.
Li, X.-H. et al., 2014. Low-threshold stimulated emission
at 249 nm and 256 nm from AlGaN-based multiple-
quantum-well lasers grown on sapphire substrates.
Applied Physics Letters, 105(14), p.141106.
Lin, B.C. et al., 2014. Design and fabrication of a InGaN
vertical-cavity surface-emitting laser with a
composition-graded electron-blocking layer. Laser
Physics Letters, 11(8), p.085002.
Martens, M. et al., 2014. Performance characteristics of
UV-C AlGaN-based lasers grown on sapphire and
bulk AlN substrates. IEEE Photonics Technology
Letters, 26(4), pp.342–345.
McIntosh, F.G. et al., 1996. Growth and characterization
of AlInGaN quaternary alloys. Applied Physics
Letters, 68(1), p.40.
Mitrofanov, O. et al., 2006. High-reflectivity ultraviolet
AlGaN⁄AlGaN distributed Bragg reflectors. Applied
Physics Letters, 88(17), p.171101.
Moe, C.G. et al., 2006. AlGaN/AlN distributed bragg
reflectors for deep ultraviolet wavelengths. Physica
Status Solidi (a), 203(8), pp.1915–1919.
Murotani, H. et al., 2011. Silicon concentration
dependence of optical polarization in AlGaN
epitaxial layers. Applied Physics Letters, 98(2),
p.021910.
Nam, K.B. et al., 2004. Unique optical properties of
AlGaN alloys and related ultraviolet emitters.
Applied Physics Letters, 84(25), pp.5264–5266.
Northrup, J.E. et al., 2012. Effect of strain and barrier
composition on the polarization of light emission
from AlGaN/AlN quantum wells. Applied Physics
Letters, 100(2), p.021101.
Pantzas, K. et al., 2012. Nanometer-scale, quantitative
composition mappings of InGaN layers from a
combination of scanning transmission electron
microscopy and energy dispersive x-ray spectroscopy.
Nanotechnology, 23(45), p.455707.
Redwing, J.M. et al., 1996. An optically pumped GaN–
AlGaN vertical cavity surface emitting laser. Applied
Physics Letters, 69(1), pp.1–3.
Reuters, B. et al., 2012. Relaxation and critical strain for
maximum In incorporation in AlInGaN on GaN
grown by metal organic vapour phase epitaxy.
Journal of Applied Physics, 112(9), p.093524.
Ryu, H.-Y. et al., 2013. Investigation of light extraction
efficiency in AlGaN deep-ultraviolet light-emitting
diodes. Applied Physics Express, 6, p.062101.
Shatalov, M. et al., 2012. AlGaN deep-ultraviolet light-
emitting diodes with external quantum efficiency
above 10%. Applied Physics Express, 5, p.082101.
Shatalov, M. et al., 2002. Deep ultraviolet light-emitting
diodes using quaternary AlInGaN multiple quantum
wells. IEEE Journal of Selected Topics in Quantum
Electronics, 8(2), pp.302–309.
BAlGaN-basedVerticalCavitySurfaceEmittingLaserOperatinginDeepUVRegion
35