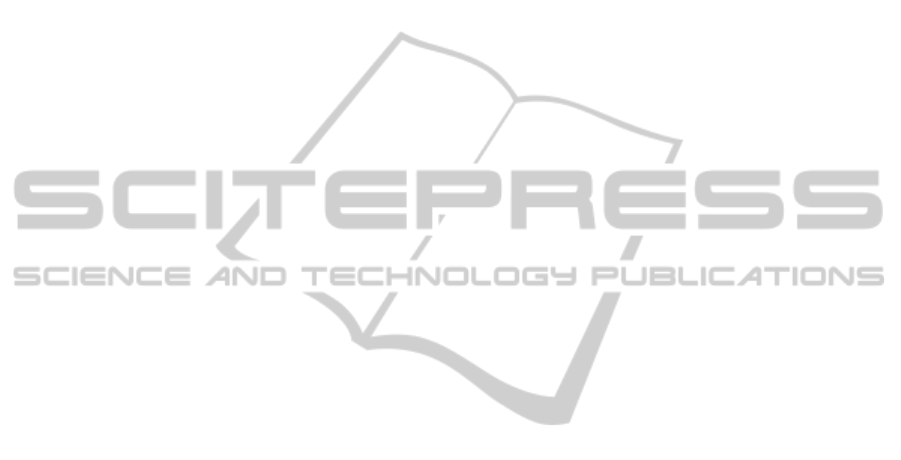
REFERENCES
D.C. Hanna et al., “Efficient and tunable operation of a
Tm-doped fibre laser,” Opt. Commun. 75, 283-286
(1990).
W. L. Barnes and J. E. Townsend, “Highly tunable and
efficient diode pumped operation of Tm3+ doped fiber
lasers,” Electron. Lett. 26, 746-747 (1990).
J.Wu, Z. Yao et al., “Highly efficient high-power thulium-
doped germinate glass fiber laser, Opt. Lett. 32, 638-
640 (2007).
S. D. Jackson, A. Sabella, and D. G. Lancaster,
“Application and Development of High-Power and
Highly Efficient Silica-Based Fiber Lasers Operating
at 2 μm,” IEEE J. of Selected Topics in Quantum
Electronics 13, 567-572 (2007).
V.V. Dvoyrin and I.T. Sorokina, “6.8W all-fiber
supercontinuum source at 1.9–2.5μm”, Laser Phys.
Lett. 11 (2014).
I.T. Sorokina, V.V. Dvoyrin, N. Tolstik, E. Sorokin, “Mid-
IR Ultrashort Pulsed Fiber-Based Lasers”, IEEE J. of
Selected Topics in Quantum Electronics, 20 , Issue: 5
(2014).
L. E. Nelson, E. P. Ippen, and H. A. Haus, “Broadly
tunable sub-500 fs pulses from an additive-pulse
mode-locked thulium-doped fiber ring laser,” Appl.
Phys. Lett. 67, 19-21 (1995).
R. C. Sharp et al.,"190-fs passively mode-locked thulium
fiber laser with a low threshold," Opt. Lett. 21, 881-
883 (1996).
Max A. Solodyankin, Elena D. Obraztsova, Anatoly S.
Lobach, Alexander I. Chernov, Anton V. Tausenev,
Vitaly I. Konov, and Evgueni M. Dianov, "Mode-
locked 1.93 μm thulium fiber laser with a carbon
nanotube absorber," Opt. Lett. 33, 1336-1338 (2008).
L. E. Nelson et al., “Ultrashort-pulse fiber ring lasers,”
Appl.Phys. B 65(2), 277–294 (1997).
C.W. Rudy, M.J.F. Digonnet, R.L. Byer1, and S. Jiang
“Thulium-doped Germanosilicate Mode-locked Fiber
Lasers”, Lasers, Sources, and Related Photonic
Devices Technical Digest, paper FTh4A.4. (2012).
V.V. Dvoyrin et al., “1.9 μm Tm
3+
- doped germanate fiber
laser source for Si-processing”, Fiber Laser
Applications (FILAS) OSA Technical Digest (2011)
paper: FThE8.
V.V. Dvoyrin, V.M. Mashinsky, L.D. Iskhakova, M.V.
Yashkov, V.F. Khopin, A.N. Gur'yanov, E.M. Dianov,
“Optical fibre with a germanate glass core for lasing
near 2µm,” QuantumElectronics 40, 1103-1105
(2010).
Q. Wang, T. Chen, K. Chen, “Mode-locked Ultrafast
Thulium fiber laser with all-fiber dispersion
management,” OSA CLEO/QELS, 2010, San Jose,
CA, paper CFK7.
K. Kieu, F.W. Wise, “Soliton Thulium-Doped Fiber Laser
with Carbon Nanotube Saturable Absorber,” IEEE
Photon. Tech. Lett. 21, 128-130 (2009).
M. Zhang, E. J. R. Kelleher, F. Torrisi, Z. Sun, T. Hasan,
D. Popa, F. Wang, A. C. Ferrari, S. V. Popov, and J.
R. Taylor, “Tm-doped fiber laser mode-locked by
graphene-polymer composite,” Opt. Express 20(22),
25077 (2012).
M. Engelbrecht et al., “Ultrafast thulium-doped fiber-
oscillator with pulse energy of 4.3 nJ,” Opt. Lett. 33,
690–692 (2008).
F. Haxsen et al., “Stretched-pulse operation of a thulium-
doped fiber laser,” Opt. Express 16(25), 20471–20476
(2008).
V. V. Dvoyrin, I. T. Sorokina, V. M. Mashinsky, L. D.
Ischakova, E. M. Dianov, V. L. Kalashnikov, M. V.
Yashkov, V. F. Khopin, A. N. Guryanov, “Tm
3+
-
doped CW fiber laser based on a highly GeO
2
-doped
dispersion-shifted fiber,” Opt. Express 19, 7992-7999
(2011).
D. Klimentov et al., Journal of Lightwave Technology 30,
1943 (2012).
K. Tamura, E. P. Ippen, and H. A. Haus, "Pulse dynamics
in stretched-pulse fiber lasers," Appl. Phys. Lett. 67,
158-160 (1995).
K. Tamura et al., "77-fs pulse generation from a stretched-
pulse mode-locked all-fiber ring laser," Opt. Lett. 18,
1080-1082 (1993).
PHOTOPTICS2015-DoctoralConsortium
86